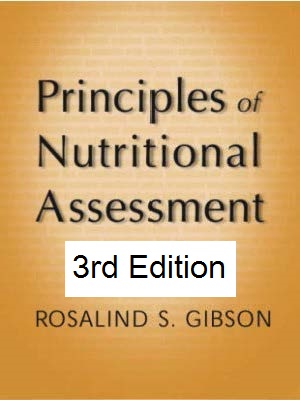
Whitfield KC, Principles of
Nutritional
Assessment:
Thiamine
3rd Edition, August 2024
Abstract
Thiamine (vitamin B1), the first vitamin discovered and synthesized, plays a critical role in energy metabolism, neurological functioning, and early cognitive development. Thiamine deficiency disorders range broadly in presentation and severity, but beriberi can be fatal. Despite its long history, and the well-established adverse outcomes of deficiency, thiamine lacks both a standardized biomarker and interpretative criteria. The most common biomarker currently in use is blood thiamine diphosphate (ThDP), likely due to the growing availability of high performance liquid chromatography equipment and ease of standardization; however the lack of clinically meaningful cut-offs limits the practical use of ThDP. Although the functional biomarker of erythrocyte transketolase activity coefficient (ETKac) is still run in a few labs and has a well-established cut-off for deficiency, the limitations of working with washed erythrocytes and the fact that this assay is not readily available in most clinical settings hinders its use. At the individual level, use of a therapeutic thiamine challenge remains the clear course of action given the safety of thiamine, as compared to known severe adverse outcomes of untreated thiamine deficiency. At the population level, challenges still exist; however, the growing interest and method development for thiamine assessments via dried blood spots is an exciting prospect. Use of dried blood spots could ease sample collection, processing, storage, and shipment costs, widening the possibility of field-friendly assessments, for example, integrated into population-level surveys in beriberi-endemic regions. Such thiamine assessments would help enormously with properly estimating the global burden of thiamine deficiency, and triggering appropriate action for public health interventions in necessary areas. CITE AS: Whitfield KC, Principles of Nutritional Assessment: Thiamine https://nutritionalassessment.org/thiamine/Email: Kyly.Whitfield@msvu.ca
Licensed under CC-BY-4.0
( PDF )
20a.1 Thiamine
The isolation and synthesis of thiamine was carried out in 1933 by Williams and Cline (1936). The structure of thiamine consists of a pyrimidine and thiazole ring linked by a methylene bridge (Figure 20a.1). Phosphorylated forms of thiamine include thiamine monophosphate (ThMP), thiamine diphosphate (ThDP) and thiamine triphosphate (ThTP), all of which can be interconverted in the tissues. Of these, ThDP is the biologically active form and accounts for nearly 90% of total thiamine in tissues (Rindi & Laforenza, 2000).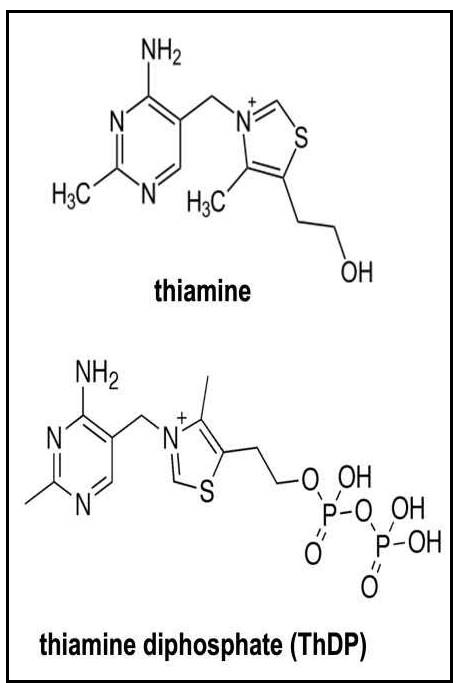
20a.1.1 Functions of thiamine
Thiamine, when converted to ThDP (shown in Figure 20a.1.1), has a major role in carbohydrate metabolism. As a co-enzyme in the pyruvate dehydrogenase complex, α‑ketoglutarate dehydrogenase complex and the branched chain α‑keto acid dehydro genase complex, ThDP is required in both the Kreb's cycle and pentose phosphate pathway (Whitfield et al., 2018). ThDP is also involved in amino acid and fatty acid metabolism. Thiamine is required for the biosynthesis of neurotransmitters (Fattal-Valevski, 2011) and in early life, thiamine has a vital role in normal cognitive development (Mimouni-Bloch et al., 2014).20a.1.2 Deficiency of thiamine in humans
Thiamine deficiency has been classically defined as either Wernicke-Korsakoff syndrome among chronic alcoholics, or beriberi: wet beriberi for cardiac presentation, dry beriberi for neurological symptoms, Shoshin beriberi for rapid onset, or infantile beriberi for pediatric presentation. However, the broad and overlapping clinical symptoms of beriberi have recently prompted use of the terms “thiamine deficiency disorders”, or “thiamine-responsive disorders” to describe patients with symptoms or disorders that improve with thiamine treatment (Whitfield et al., 2018). Thiamine deficiency disorders have a broad clinical presentation (see examples in Table 20a.1 ), often leaving the disorder unrecognized, or causing mis-diagnoses (Smith et al., 2020).Organ system | Select manifestations of thiamine deficiency |
---|---|
Cardiorespiratory |
* lower extremity edema (adults) or generalized edema (infants) * pulmonary hypertension * right-sided heart failure * tachycardia * tachypnea |
Gastrointestinal |
* anorexia * constipation * diarrhea * vomiting |
Musculoskeletal |
* muscle atrophy * peripheral neuropathy * weakness |
Nervous |
* ataxia * encephalitic presentations (brain inflammation) * impaired cognitive development (language, motor delays) * nystagmus * ophthalmoplegia |
- increased thiamine requirements, caused by fever, sepsis, malignancy, etc.;
- increased thiamine losses, caused by hemodialysis and peritoneal dialysis, chronic diuretic use, prolonged vomiting or diarrhea, etc.; and/or
- decreased thiamine intake and/or absorption, caused by malnutrition, alcoholism, hyperemesis gravidarum, consumption of thiamine antagonists or thiaminases, etc.
It is well-established that thiamine requirements increase with higher carbohydrate intakes and greater energy expenditures (Elmadfa et al.,2001). For example, periodic outbreaks of thiamine deficiency have been reported among populations consuming monotonous rice-based diets such as illegal gold miners in French Guiana (Mosnier et al., 2017), Thai commercial fishermen (Doung-ngern et al., 2007), and Gambian farmers (Thurnham et al., 2011).
Given the role of thiamine and mechanisms of deficiency highlighted above, individuals with clinical conditions such as patients with chronic renal failure (Talwar et al., 2000), malnutrition (Hiffler et al., 2016), people living with HIV (Müri et al., 1999) and those with critical illnesses (Mates et al., 2021) may be at risk of deficiency.
The most common clinical presentation of thiamine deficiency in high-income settings is among chronic alcoholics due to general malnutrition and alcohol-induced increases in thiamine requirements and impaired thiamine absorption (Latt & Dore, 2014). Wernicke's encephalopathy is an acute, reversible presentation of thiamine deficiency that can lead to Korsakoff syndrome; the often-overlapping presentation is known as Wernicke-Korsakoff syndrome (Latt & Dore, 2014). Treatment of Wernicke-Korsakoff syndrome with both thiamine and magnesium has been recommended by Peake et al. (2013) because magnesium is also required for the full activation of the transketolase enzyme in the pentose phosphate pathway.
Another key risk group is post-operative bariatric surgery patients, in whom thiamine deficiency is caused either by lower dietary intakes or thiamine malabsorption due to the bypass or removal of the proximal small intestine (Frank, 2015). In addition, Mates et al. (2021) recently hypothesized that thiamine deficiency may be more common, yet unrecognized, among older adults in high-income settings presenting with weakness, falling, delirium, and gastrointestinal symptoms. Thiamine deficiency in older adults could be caused by the co-existence of chronic disease burden (i.e. inflammation), insufficient thiamine intake due to age-related malnutrition, and increased thiamine losses (i.e., higher diuretic use in this population) (Mates et al., 2021). Two ongoing areas of clinical research include use of thiamine, or the highly bioavailable analogue benfotiamine, in the treatment of both Alzheimer's Disease and/or dementia (Gibson et al., 2016; Pan et al., 2016; Sambon et al., 2021) and diabetic polyneuropathy (Stracke et al., 2008).
Another growing area of interest is the impact of early life thiamine intake on cognitive development. An unfortunate “natural experiment” took place in Israel in 2003 when thiamine was erroneously excluded from infant formula, resulting in otherwise well-nourished infants presenting with symptoms of thiamine deficiency (Fattal-Valevski et al., 2005). Infants with sub-clinical infantile beriberi were followed up and later experienced delays in language (Fattal-Valevski et al., 2009) and motor skills development (Harel et al., 2017). This is relevant as the thiamine content of human milk depends on maternal thiamine intake (Dror & Allen, 2018). Thus, exclusively breastfed infants of mothers with low thiamine intakes could be at risk of either mortality via infantile beriberi, or impaired cognitive development, despite no signs of clinical deficiency. Empirical studies are currently underway to better understand the impact of thiamine exposure during the first six months of life on various aspects of cognitive and neurological development (Whitfield et al., 2019). Results thus far support that thiamine is integral to normal expressive and receptive language development (Measelle et al., 2021).
20a.1.3 Food sources and dietary intakes
Whole grain cereals, pork, and legumes are the richest food sources of thiamine, followed by other meats, fish, green vegetables, fruits and milk. Polished rice, sugar, alcohol, fat and other refined foods are poor sources of thiamine. Thiamine is thought to be highly bioavailable; notable losses would occur only with concurrent consumption of known thiamine antagonists such as betel nuts or tea, or thiaminase-containing raw fish, ferns, or African silkworm larvae (Vimokesant et al., 1982; Whitfield et al., 2018).While whole grains are a good source of thiamine, milling induces substantial losses because most thiamine is stored in the bran; up to 50% of thiamine in wheat is lost during milling (Oghbaei & Prakash, 2016). Consequently, thiamine enrichment or fortification programs contribute greatly to thiamine intakes globally (Whitfield et al., 2021). Besides mandatory fortification of wheat flour and rice, which have been in place in Canada, the United States, and the Philippines since the 1940s (Aykroyd et al., 1945; Salcedo & Pedroche, 1949), the voluntary fortification of ready-to-eat breakfast cereals also contributes meaningfully to thiamine intakes (Fulgoni (III) & Buckley, 2015). Thiamine-rich yeast in leavened products also contributes to thiamine intake, in addition to fortified flour: Australian researchers reported that despite heat-induced baking losses, baked bread contained more thiamine than the fortified wheat flour from which it was made (Tiong et al., 2015).
Thiamine is prone to degradation by exposure to UV light, heat, oxygen and moisture (Camire et al., 1990). Of these factors, pH has the greatest effect on thiamine stability, with even weak alkaline conditions leading to thiamine degradation (Lešková et al., 2006). Thiamine is rapidly destroyed at elevated temperatures unless the pH is below 5. Hence, the addition of sodium bicarbonate to green vegetables to retain their green color destroys thiamine (Aughey and Daniel, 1940). Thiamine is lost when cooking water is discarded because it is a water-soluble vitamin. In meat thiamine is leeched into cooking juices, although losses are generally lower in dark muscles, possibly due to a protective effect from their fat content (Lešková et al., 2006). In alkaline solution, thiamine may be oxidized to the fluorescent compound thiochrome. This reaction is widely used for measuring thiamine in biological tissues and fluids.
20a.1.4 Effects of high intakes of thiamine
No adverse effects of excessive thiamine intakes have been described, except some minor transient local irritation or generalized pruritus at high treatment doses (100mg intravenous administration) (Wrenn et al., 1989). The relatively few studies conducted on the adverse effects of large doses of thiamine were insufficient to allow a Tolerable Upper Intake Level (UL) to be set by the National Academy of Medicine, formerly the Institute of Medicine (Institute of Medicine, 1998). Likewise, the European Food Safety Authority (EFSA) did not set a UL for thiamine, and concluded that the current levels of intake from thimaine from all sources do not represent a health risk for the population (EFSA 2016).20a.1.5 Pharmacokinetics of thiamine supplementation
Oral thiamine supplementation results in a rapid increase in blood thiamine markers (Smithline et al., 2012). Thiamine is absorbed in the proximal small intestine via passive diffusion, or with an active, carrier-mediated transport mechanism at low concentrations. Some researchers have reported that, in a single dose, no more than between 2.5 to 8.3mg is absorbed (Morrison & Campbell, 1960; Thomson & Leevy, 1972). A more recent pharmacokinetics study with 12 healthy American participants found that the thiamine absorption mechanism was not saturable with a single high oral dose of 1,500mg thiamine hydrochloride (Smithline et al., 2012). However, most hospitals would be unlikely to prescribe such a high dose; 100mg thiamine supplements are much more common (Smith et al., 2021). In a population at high-risk to thiamine deficiency in Cambodia, Coats et al. (2013) found that 16 lactating women also showed a rapid blood (and breastmilk) thiamine response to both a single 100mg dose, as well as 5 consecutive days of 100mg doses of thiamine hydrochloride.20a.2 Biochemical indices of thiamine status
Despite thiamine's long history, there is still debate around the best biochemical biomarker to define status (Pfeiffer et al., 2021; Whitfield et al., 2018). The two most common biochemical indices of thiamine status are the direct measure of thiamine diphosphate (ThDP) in either whole blood or erythrocytes, or the functional measure that assesses the degree of saturation of the ThDP-dependent enzyme transketolase, the erythrocyte transketolase activity coefficient (ETKac). Thiamine in erythrocytes depletes at approximately the same rate as other tissues (Brin, 1964), making blood an ideal proxy for thiamine status. Assessment of thiamine from dried blood spots is a growing research area. Other less commonly employed measures, serum/plasma thiamine and urinary thiamine excretion, will also be outlined briefly below.Regardless of measurement (ThDP or ETKac), blood samples should be collected into blood tubes containing an anticoagulant (i.e. heparin or EDTA). Samples are stable at room temperature for a few hours, at −20°C for a few weeks, and −70°C for several months to years. Freeze-thaw cycles should be avoided (Whitfield et al., 2018). If analyses are to be completed in erythrocytes, then care must be taken to wash cells before freezing. Samples should be frozen completely (i.e. overnight at −70°C) to ensure complete lysis of erythrocytes (Lu & Frank, 2008).
20a.2.1 Thiamine diphosphate in whole blood or erythrocytes
The direct assessment of thiamine diphosphate (ThDP) in either whole blood or washed erythrocytes is currently the most common method of assessing thiamine status, even though this direct measurement does not reflect metabolic function (Whitfield et al., 2018). ThDP (sometimes along with thiamine and thiamine monophosphate (ThMP)) is most often determined via reverse-phase high performance liquid chromatography with a fluorescence detector (HPLC-FLD). A derivatizing agent (i.e. potassium ferricyanide) is used to alter the molecular structure of thiamine to thiochrome (three rings rather than two rings of thiamine); it is this three-ring structure that fluoresces and is detected. Two detailed methods are published (Lu & Frank, 2008; Mancinelli et al., 2003). Note that mass spectrometry (MS)-based methods (e.g., liquid chromatography (LC)-MS/MS) have also been developed, which allow for measurement of underivatized ThDP. However, this equipment tends to be more expensive and less commonly available than HPLC-FLD (Whitfield et al., 2018).Erythrocyte ThDP (eThDP) and whole blood ThDP have been reported to correlate well (r=0.97) (Talwar et al., 2000), so many researchers opt to utilize whole blood samples to reduce the time and effort in sample processing of washed erythrocytes (Lu & Frank, 2008). If erythrocytes are used, cells should be washed three times in phosphate buffered saline to avoid osmotic damage to the cells (Whitfield et al., 2018), and care should be taken to remove all buffy coat as leukocytes are rich in thiamine (Chu and Hall, 1990).
Best practice is for whole blood measures to be reported in the context of hematocrit (nmol/L erythrocytes) or hemoglobin (nmol/g hemoglobin) for consistency across studies and so that whole blood and eThDP can be compared (Whitfield et al., 2018).
Interpretative criteria
There is currently no agreed upon cut-off to define risk of deficiency (Whitfield et al., 2018). Although a wide range of cut-offs have been employed, these cut-offs are often statistically derived and thus do not necessarily align with clinical signs of thiamine deficiency disorders. See Table 20a.2 for some examples of cut-offs previously used for eThDP.
Thiamine status cut- offs lower than reference range | Reference(s) | Description |
---|---|---|
< 180nmol/L |
Mancinelli et al., 2003 |
25th percentile of n=103 (45 men and 58 women) healthy controls, employees of University “La Sapienza” Hospital, Rome, Italy |
< 165nmol/L | Baines, 1985 |
Lower bound of 95% reference range (165–286 nmol/L) n=48 (25 men and 23 women) healthy staff at Broadgreen Hospital, Liverpool, UK |
< 150nmol/L |
Warnock, et al., 1978 |
Lower bound of normal range (50–150ng/mL packed cells) from n=21 healthy adults (Warnock, et al., 1978) Note that this cut-off was also employed in both Bailey et al., 1997 and Bailey et al., 1994 where authors noted 50ng/mL ≈ 148.2 nmol/L |
< 150nmol/L |
Coats et al., 2012 |
Reference range of 80–150nmol/L for whole blood ThDP, or 150–290nmol/L for eThDP equivalent (ThDP corrected for hematocrit) |
< 140nmol/L |
Wilkinson et al., 1997 |
Lower limit of normal eThDP (cut-off of lowest 2.5%) of healthy blood donors in Christchurch, New Zealand; n unknown |
< 135nmol/L |
Floridi et al., 1984 |
eThDP reference range of 135–330nmol/L among n=33 healthy Italian volunteers (18–50y) |
Marginal thiamine deficiency | Reference | Description |
< 120–150nmol/L |
Bailey and Finglas, 1990 | Cut-off reported, but details unknown |
Thiamine deficiency | Reference | Description |
< 120nmol/L |
Bailey and Finglas, 1990 | Cut-off reported, but details unknown |
< 120nmol/L |
Bailey et al., 1994 |
eThDP < 118.5nmol/L was used to categorize thiamine deficiency |
At the population level, a consensus eThDP cut-off still needs to be established. This is a clear and pressing research question, as population-level risk varies widely depending on the cut-off employed. For instance, eThDP was measured in the National Micronutrient Survey linked to the Cambodian Demographic and Health Survey (2014) and suboptimal thiamine status ranged from 27% to 78% in women of reproductive age and 15% to 58% in children aged 6-69 months, depending on which cut-off was employed; see Table 20a.3 (Whitfield et al., 2017). The Global Thiamine Alliance has called for the development of a clinically relevant thiamine cut-off (Bourassa et al., 2021; Whitfield et al., 2018). Comparison of ETKac and eThDP revealed an inflection point at an ETKac of 1.25 (indicating deficiency) with a ThDP of 270 ng/g hemoglobin (Talwar et al., 2000). This converts to approximately 224nmol ThDP/g hemoglobin. However, this comparison was made among a relatively small group of 63 medical and surgical patients in the United Kingdom thought to be at risk of thiamine deficiency (Talwar et al., 2000). Hence, it is unclear whether this cut-off would be valid among populations with endemic thiamine deficiency (Whitfield et al., 2018).
Thiamine status eThDP, nmol/L | Women aged 15–49y, n=719 |
Children aged 6–69mos, n=761 |
---|---|---|
Mean (95% CI) | 150 (146–153) | 174 (171–178) |
Range | 41–352 | 66–379 |
Thiamine status cut-offsa | ||
Lower than reference range | ||
< 180nmol/L | 558 (78%) | 441 (58%) |
< 165nmol/L | 481 (67%) | 347 (46%) |
< 150nmol/L | 398 (55%) | 271 (36%) |
< 140nmol/L | 336 (47%) | 217 (29%) |
< 135nmol/L | 292 (41%) | 188 (25%) |
Marginal thiamine deficiency | ||
120 – 150nmol/L | 208 (29%) | 158 (21%) |
Thiamine deficiency | ||
< 120nmol/L | 192 (27%) | 114 (15%) |
Factors affecting whole blood or erythrocyte ThDP
Concern has been raised about the use of the direct assessment of ThDP in blood samples in populations with persistently low thiamine intakes, such as groups in low- and middle-income countries heavily reliant on white, polished rice and likely also at risk of chronic inflammation and/or frequent infection. This concern is highlighted in a case-control study in rural Cambodia that found no differences in whole blood ThDP (corrected for hematocrit) between breastfed infants with and without clinical signs of beriberi and the mothers of the cases and controls (Coats et al., 2012). The means (95% CI) ThDP/Hct (i.e.,corrected ThDP) for infant cases and controls were 135 (114–157) and 159 (133–184nmol/L) erythrocytes (p=0.08), respectively. For mothers of cases and controls, authors reported 141 (126–158) and 150 (124–166nmol/L) erythrocytes (p=0.46), respectively (Coats et al., 2012). These results call into question whether ThDP is a valid biomarker of thiamine status.20a.2.2 Erythrocyte transketolase activity coefficient (ETKac)
Transketolase (EC 2.2.1.1) is a ThDP-dependent enzyme. Measurement of the activity of this enzyme in erythrocytes is commonly used as an index of thiamine nutritional status. The principle of this assay is to assess the saturation of the ThDP-dependent enzyme transketolase by measuring enzyme activity before and after the addition of excess exogenous ThDP.In outline, this assay and similar procedures are used for parallel assays for riboflavin and pyridoxine and involves the following stages:
- The basal activity of the enzyme (transketolase) in erythrocytes is measured. This represents the endogenous enzyme activity and depends on the amount of the coenzyme (ThDP) in the erythrocytes.
- The enzyme activity with excess coenzyme added in vitro is then determined. This equates to the maximum potential enzyme activity and is referred to as total or “stimulated” activity.
- The basal and enzyme activities are then compared to indicate the degree of unsaturation of the enzyme with the coenzyme.
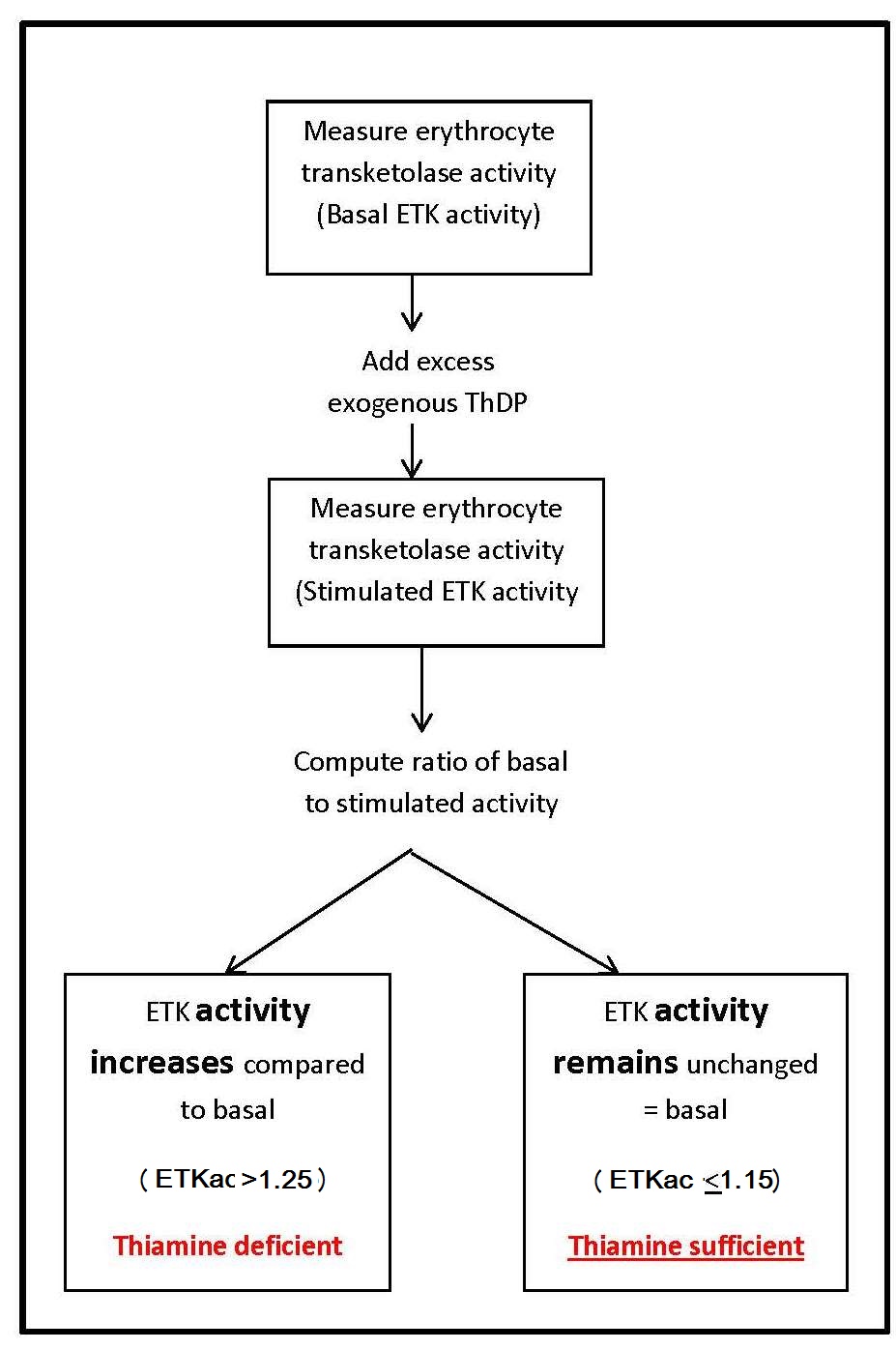
Sometimes the percentage of stimulation, also known as the percentage activation α, is calculated from the activity coefficient as (ETKac × 100) − 100. This is known classically as the thiamine pyrophosphate (diphosphate) effect, “TPP effect”. If not reported as a ratio (ETKac), the basal and stimulated enzyme activities can be expressed per gram of hemoglobin, per number of erythrocytes, or in terms of the volume of erythrocytes (in mL).
Based originally on methods of Brin (1966) and later Smeets et al. (1971) and Vuilleuimier et al. (1983), this method has been recently updated for analysis on a plate reader with a more convenient 96-well plate configuration (Jones et al., 2021). An important consideration for this 96-well format in particular is the need for identical temperature exposure to all wells: the plate reader's incubator must be functioning well to maintain analytical temperatures (Whitfield et al., 2018).
ETKac Interpretive criteria
The interpretative criteria for ETKac, shown in Table 20a.4 , are consistent throughout the literature (Institute of Medicine, 1998; Ruston et al., 2004; Turck et al., 2016; World Health Organization, 1999).ETKac and α activation | Status |
---|---|
ETKac < 1.15, or α < 15% | adequate thiamine status |
ETKac 1.15–1.25, or α 15–25% |
thiamine insufficiency / marginal thiamine deficiency |
ETKac > 1.25, or α > 25% | thiamine deficiency |
Factors affecting erythrocyte transketolase activity
In thiamine deficiency, the basal level of erythrocyte transketolase activity is low and an enhancement in the enzyme activity after the addition of ThDP in vitro is generally observed. Prolonged thiamine deficiency, however, induces a reduction in the apotransketolase enzyme level. Consequently, both the basal and stimulated erythrocyte transketolase activities tend to fall, with no change in the percentage activation α, giving rise to misleadingly “normal” ETKac values (Schrijver, 1991). In such cases, the basal enzyme activity should also be considered (Nixon et al., 1990; Soukaloun et al., 2011).Use of the ETK ratio versus basal activity. The ratio of stimulated to basal enzyme activity is used as the primary measure because (a) the inter-subject variation in basal erythrocyte enzyme activity is large, and (b) it is assumed that apoenzyme levels are not affected by vitamin deficiencies. This latter assumption may not be correct, however (Schrijver, 1991). Vitamin deficiency or excess and other factors such as the presence of certain diseases and the administration of hormones and drugs, may affect apoenzyme levels and confound the interpretation (Talwar et al., 2000). Hence, when interpreting the results, it is advisable to take into account the basal enzyme activity, as well as its activation with coenzyme (Bamji, 1981). Jones et al. (2020) argue that use of the ratio reduces the need for the precise assay conditions such as the optimal path length, which is required when expressing basal and stimulated activities alone (Bates, 1997). Conversely, Soukaloun et al. (2011) specifically recommend interpretation of basal ETK rather than the ratio ETKac among infants. They argue that, due to low thiamine exposure in utero and postnatally, apoenzyme may be unstable and concentrations may fall. As a result, ETKac could underestimate thiamine deficiency in infancy.
Age of the erythrocytes influences enzyme activity; levels decline as the cells age. As a result, the basal enzyme activity depends on the mean age of the erythrocytes (Thurnham, 1981). Hence, in patients undergoing treatment for iron deficiency, for example, the basal level of erythrocyte transketolase activity will be increased as a result of the reticulocytosis (Thurnham, 1981).
Age affects transketolase activity. Older individuals tend to have lower activities, possibly as a result of a defect in the apoenzyme rather than inadequate activity of the coenzyme (O'Rourke et al., 1990).
Certain disease states are associated with low erythrocyte transketolase activity (Talwar et al., 2000). In some cases (e.g., diabetes and liver disease), the reduced activity results from a reduced apoenzyme level associated with the disease. In other diseases (e.g., certain cancers), the percentage activation α is enhanced despite adequate thiamine intakes because the conversion of thiamine to ThDP is impaired (Basu & Dickerson, 1976). Patients with polyneuritis, uremic neuropathy, and disorders of the gastrointestinal tract, also have low transketolase activity (Kjøsen & Seim, 1977), although the cause is uncertain.
Intra-subject variation in erythrocyte transketolase activity can be large, even in individuals with fixed thiamine intakes (van Dokkum et al., 1990). Such large variations occur because individuals differ in their sensitivity to thiamine deficiency. This leads to difficulties in interpreting transketolase activity coefficients when values are indicative of adequacy (i.e., 1.0) and those indicative of thiamine deficiency (i.e., ≥ 1.25). Hence, correlation of ETKac and intakes within this range may be poor (Vir & Love, 1979).
In view of these uncertainties, it is perhaps not surprising that ETKac may not correlate significantly with thiamine intakes. In general, such relationships have only been reported in surveys of population groups known to be vulnerable to thiamine deficiency, such as the elderly. Correlations, however, are less obvious in groups such as adolescents who are more likely to have adequate thiamine intakes (Bailey et al., 1994). More recent studies that have measured transketolase activity generally do not report correlations between dietary thiamine and transketolase activity (2011). An exception is a study in Laos, where postpartum restrictive diets are common, when Khounnorath et al. (2011) surpisinly reported lower basal transketolase activity among infants of mothers who had eaten pork since delivery, a good source of thiamine. Although a number of studies have found increased whole blood or erythrocyte ThDP concentrations after thiamine supplementation (e.g., (Coats et al., 2013; Smithline et al., 2012), few studies have examined ETKac in the context of thiamine supplementation. Gallant et al. (2021) recently reported significantly lower ETKac among lactating women consuming a daily, low-dose thiamine supplement (between 1.2 and 10mg/d) over 22 weeks, as compared to a placebo; also see Table 20a.5 However, higher dose supplementation (100mg/d) among 25 Karen mothers in the Mae La refugee camp on the Thai-Myanmar border did not significantly impact ETKac when compared to the response of 22 unsupplemented mothers (McGready et al., 2001).
Likewise, abnormal erythrocyte transketolase activity may not be associated with clinical signs of thiamine deficiency disorders. For example, in the same study of Karen refugees, symptoms such as paresthesia were not associated with abnormal erythrocyte transketolase activity at 30 wk gestation or at delivery (McGready et al., 2001). However, both basal transketolase activity and ETKac differed significantly between infants with clinical beriberi (n=47) and controls (n=47 febrile controls and n=47 afebrile controls) in a Laos study (2011).
The ETKac assay is often critiqued for poor inter-assay precision, as well as a lack of standardization (Whitfield et al., 2018) and challenges with inter-laboratory comparisons (Bailey et al., 1994). More recently, the limited availability of the ETKac assay has prevented widespread use, particularly in clinical, remote, or low-income settings (Barennes et al., 2015; Sastry et al., 2021). It appears that only a few laboratories in Scotland and England still actively assess ETKac (Jones et al., 2021; Maguire et al., 2021). Two examples of ETKac from a low- and high-risk population, without and following thiamine supplementation are shown in Table 20a.5.
Population | Participant group | n | ETKac (mean ± SD) | ETKac > 1.25(%) |
---|---|---|---|---|
United Kingdom | 4–10y | 101 | 1.10 ± 0.05 | 0% |
11–18y | 164 | 1.12 ± 0.05 | 0% | |
19–64y | 513 | 1.11 ± 0.05 | 1% | |
≥ 65y | 153 | 1.10 ± 0.06 | 1% | |
Cambodia | 2 weeks postpartum, before any thiamine supplementation | 334 | 1.16 ± 0.09 | 15% |
24 weeks postpartum (placebo group) | 83 | 1.20 ± 0.08 | 25% | |
24 weeks postpartum, after 22 weeks of thiamine supplementation: 1.2mg/d | 86 | 1.15 ± 0.06 | 7% | |
24 weeks postpartum, after 22 weeks of thiamine supplementation: 2.4mg/d | 81 | 1.14 ± 0.07 | 4% | |
24 weeks postpartum, after 22 weeks of thiamine supplementation: 10mg/d | 85 | 1.12 ± 0.06 | 5% |
20a.2.3 Thiamine assessment with dried blood spots
Although blood is an ideal medium for assessing thiamine status (Brin, 1964; Whitfield et al., 2018), there are several challenges with venipuncture, particularly in low-income settings. It is an invasive procedure that requires clinical expertise (trained phlebotomists), facilities to process samples (refrigerated centrifuge if washing erythrocytes) and a cold chain Huang et al., 2020; Verstraete & Stove, 2021b). For infants in particular, the volume of blood, invasiveness of venipuncture, and the need to hire pediatric phlebotomists, may lead to challenges with traditional venous blood collection. The utilization of dried blood spots addresses many of these challenges, simplifying both sample collection and storage. Dried blood spots would allow fingerprick capillary blood collection to replace venipuncture and there is potential for ambient, rather than −80°C freezer, storage (Verstraete & Stove, 2021b). Even if cold chain transport is required, dried blood spots are much smaller and pose a lower biohazard risk, than aliquots of frozen blood.A few different methods employing dried blood spot sample collection have been published in recent years. Mathew et al. (2019) validated a method using HPLC-FLD. However, the method requires a known volume of venous blood pipetted onto the filter paper cards, limiting its practical use. Huang et al. (2020) also developed a HPLC-FLD method, while Verstraete and Stove's method uses LC-MS/MS (Verstraete & Stove, 2021a).
In 2020, a method to assess ThDP, as well as thiamine and ThMP from capillary dried blood spots was developed by Huang et al.(2020). Authors collected venous whole blood samples (stored at −80°C until analysis), as well as capillary fingerprick samples (stored at −20°C until analysis), from 20 healthy Australian volunteers. There was a strong linear correlation between the results for venous whole blood ThDP and capillary dried blood spot ThDP (r=0.9636; p< 0.001). ThDP coefficients of variation (%CVs) for low and high quality controls were: intra-day: 3.5% and 2.7%, respectively; inter-day: 4.3% and 3.0%, respectively. Nevertheless, the ThDP measured in capillary dried blood spots was 6.4% higher as compared to venous whole blood, which authors speculated could be attributed to the higher hematocrit values of capillary samples. Hence, although promising, this method has a few limitations. While sample extraction from the filter paper cards was completed with a standard 6.35mm punch disc, a practice intended to minimize the need for hematocrit assessment, a limitation of this method is the unknown sample volume. In addition, cold chain storage is likely to still be required for this method: although ThDP appeared to be stable when dried blood spot cards were stored at −20°C, preliminary analyses showed significant losses during ambient storage (Huang et al., 2020).
As noted above, a standard sample volume or hematocrit assessment is essential because ThDP is found mainly in erythrocytes (Whitfield et al., 2018). However, Verstraete and Stove recently reported a novel method employing volumetric absorptive microsampling (VAMS), wherein a collection device with a plastic handler and polymeric tip absorbs a fixed volume of blood, thus negating the need for additional hematocrit assessment (Verstraete & Stove, 2021b). Authors reported good agreement between venous whole blood and capillary VAMS samples, with a mean bias of −1.0% (95% CI: −4.1%, 2.0%) which was not significantly different from zero. The %CVs were 6.5% and 2.2% for capillary VAMS and venous whole blood samples, respectively. “Uncontrolled transport” was also assessed by sending capillary VAMS samples through the regular mail, thus subjecting samples to 2–5d of ambient conditions. These “uncontrolled” samples had a mean difference of 1.0% (95% CI: −18.6%, 20.6%) and 1.0% (95% CI: −14.7%, 16.7%) in two analyses when compared to “controlled” capillary VAMS samples that had been stored at −80°C until analysis. Although only 3% of samples exceeded a 20% difference threshold set to compare “controlled” and “uncontrolled” samples, the wide 95% CI is of concern and cold chain transport and storage may still be warranted. This is particularly true as these “controlled” samples were mailed during the Belgian winter, when ambient temperatures were likely low (Verstraete & Stove, 2021b). However, during method development Verstraete and Stove reported that VAMS samples were stable for 1 month at room temperature, or for 1 week at either 60°C or at 80% humidity (Verstraete & Stove, 2021a).
A strength of this method is the vastly simplified sample collection and known sample volume. Although promising, this method comparison was completed among 50 healthy Belgian volunteers with relatively high ThDP concentrations. As such, method assessment among participants with lower thiamine status and with health profiles more common in beriberi- endemic regions (e.g. higher inflammation, genetic hemoglobin disorders, environmental enteric dysfunction, malaria, tuberculosis, HIV, etc) should be undertaken.
Interpretive criteria
To date, published work has focussed on method development and comparisons; no thiamine assessments have been completed at large scale with dried blood spot samples. As a result, appropriate cut-offs have not yet been established. Although the same HPLC-FLD or LC-MS/MS methods are employed as those used for venous samples, the established differences between venous and capillary blood should be taken into account when a cut-off is established. In both infants and adults, capillary blood samples have higher hematocrit and red blood cell counts than venous samples (Kayiran et al., 2003), so that cut-offs may need to be lower if capillary samples are collected.20a.2.4 Serum or plasma thiamine
Serum or plasma thiamine assessments with either HPLC-FLD or LC-MS/MS are available in both clinical (Mates et al., 2021) and research settings (McCann et al., 2017). McCann et al. (2017) compared plasma total thiamine (thiamine + ThMP) to eThDP among 196 rural Cambodian women of reproductive age before and after a 6mos ad libitum thiamine-fortified fish sauce intervention. They found a moderate, significant correlation between measures (baseline: ρ=0.554, p < 0.01; post-thiamine intervention: ρ=0.60, p < 0.01) (McCann et al., 2017). However, the use of serum or plasma thiamine concentrations to assess thiamine status is generally not advised, as these assessments often measure free thiamine or ThMP, which are not reflective of total body stores (Mates et al., 2021). In addition, serum or plasma would only reflect recent intake rather than true tisssue thiamine status (Whitfield et al., 2018). Serum or plasma thiamine could also be impacted by various disease states such as acute hepatic injury and is thought to be transiently decreased in critically ill patients after trauma or those experiencing sepsis (Frank, 2015).20a.2.5 Urinary thiamine excretion
Urinary thiamine is no longer a common method of thiamine assessment. Collection of 24h urine samples is logistically challenging and imposes a high participant burden. Although casual urine samples can be collected, when thiamine excretion is expressed per g creatinine, urinary thiamine is still not the best indicator of status. For instance, urinary thiamine excretion did not correlate well with ETKac, or with clinical signs of deficiency among a group of 79 alcoholic patients in Australia (Wood et al., 1977).In principle, among thiamine-replete individuals, when the main tissue compartment is saturated and the urinary excretion threshold is exceeded, thiamine levels in the urine do not generally reflect body stores; instead, they parallel recent dietary intakes. In contrast, when the tissues are unsaturated, excretion is limited because the body conserves thiamine. When dietary intakes are sufficient, for example 0.5mg thiamine per 1,000kcal, approximately 100µg thiamine is excreted (Sauberlich, 1967). At lower intakes, however, urinary excretion of thiamine is no longer linearly related to intake (Oldham, 1962). This critical point is thought to be around intakes of 0.2mg thiamine per 1,000kcal, yielding urinary thiamine excretions between 5–20µg thiamine, with clinical beriberi cases yielding excretions of 0–15µg thiamine (Sauberlich, 1967). In addition, at low thiamine intakes, most of the thiamine excretion is in the form of metabolites such as pyramin, an even less sensitive measure than urinary thiamine (Sauberlich, 1967).
The intra-subject variability for urinary thiamine excretion based on a single 24h urine sample is high, with %CVs ranging from 11% to 13%, even for participants on a constant diet for 60d (van Dokkum et al., 1990). Furthermore, urinary thiamine excretion is increased by the use of certain drugs, particularly diuretics (Suter & Vetter, 2000). In addition, age-specific interpretive criteria are likely to be necessary as children have higher levels of thiamine excretion than adults when expressed on a per g creatinine basis (van Dokkum et al., 1990).