McNulty H1,
Principles of Nutritional
Assessment: Folate
3rd Edition, August 2024
Abstract
The function of folate, in its various co-factor forms, is in mediating one-carbon metabolism, a network of pathways involving the transfer and utilization of single-carbon units, including methylene, forminino, methyl, methenyl, and formyl groups. Folate is thus essential for key biological functions, including the biosynthesis of DNA, serine and glycine metabolism, and methionine synthesis. Folate, in the form of 5‑methyltetrahydrofolate, along with vitamin B12 (as methylcobalamin), is required for the synthesis of methionine from homocysteine, and in turn the generation of S‑adenosylmethionine, a methyl group donor used in numerous reactions, including the methylation of DNA, RNA, proteins, and phospholipids. Clinical deficiency of folate is manifested as megaloblastic anemia, characterized by abnormal cell replication in the hematopoietic system, megaloblasts in the bone marrow and macrocytes in the peripheral blood. The megaloblastic anemia of folate deficiency is identical to that of vitamin B12 deficiency, and specific biomarker testing is essential to provide a differential diagnosis. Folate-related anemia occurs commonly in pregnant and lactating women in low- and middle-income countries. Clinical folate deficiency is less common in high-income countries, but subclinical deficiency is widespread, especially in women of reproductive age and in the presence of certain diseases and drugs. Notably, maternal folate nutrition before and in early pregnancy plays a critical role in fetal development, with conclusive scientific evidence that folic acid supplementation in early pregnancy protects against the occurrence of neural tube defects. Serum and red blood cell (RBC) folate are the biomarkers used to assess folate status, whilst plasma homocysteine provides a functional indictor of status. CITE AS: McNuty H, Principles of Nutritional Assessment: Folate https://nutritionalassessment.org/folate/Email: h.mcnulty@ulster.ac.uk
Licensed under CC-BY-4.0
( PDF )
22a.1 Folate
Folate is a generic term referring to both natural folates and folic acid (pteroylmonoglutamic acid, PGA), the synthetic form used in supplements and fortified food. All folate forms comprise three moieties: a pteridine; a p‑aminobenzoic acid (PABA) and a glutamate residue (Figure 22a.1).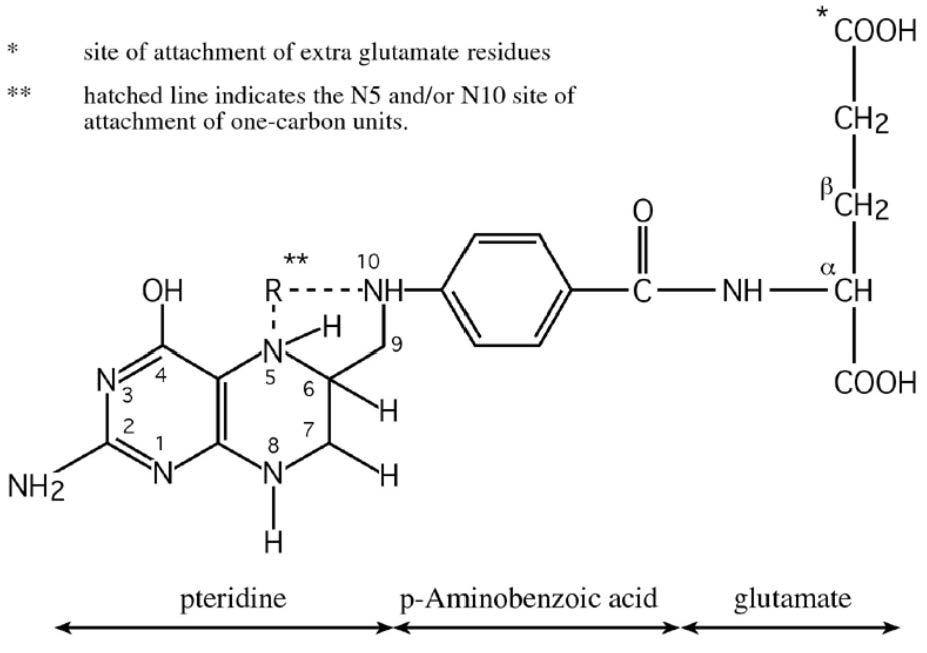
22a.1.1 Functions of folate
Folate coenzymes are required for one-carbon metabolism involving the transfer and ulilization of single-carbon atom units, including methylene (CH2), forminino (CH=NH), methyl (CH3), methenyl (CH), and formyl (CHO) groups (Figure 22a.2).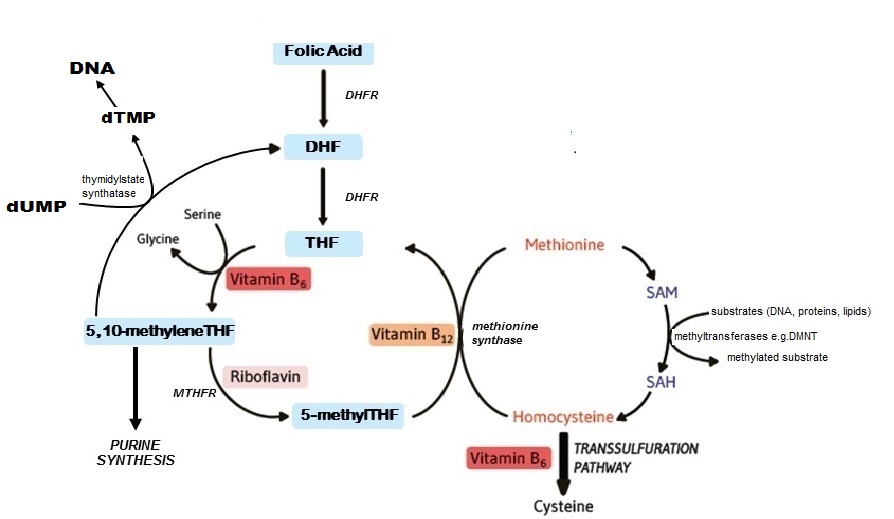
22a.1.2 Deficiency of folate in humans
Severe deficiency of folate leads to megaloblastic anemia, presenting as fatigue, weakness, and shortness of breath owing to a low red blood cell count. This condition is hematologically characterized by the presence of immature, enlarged nucleated cells, reflecting impaired DNA synthesis as a result of folate depletion. Rapidly proliferating cells are especially sensitive to abnormalities in DNA synthesis. Hence, the manifestations of folate deficiency appear first in the hematopoietic system and then in the epithelial cell surfaces and the gonads. Abnormal cell replication in the hematopoietic system, manifested by hypersegmented neutrophils, is one of the earliest morphological changes. Later, megaloblasts appear in the bone marrow and macrocytes in the peripheral blood. Some additional signs and symptoms that have been reported with clinical folate deficiency include fatigue, angular cheilosis, anorexia, insomnia, glossitis, recurrent aphthous ulcers, and pallor of the skin and mucous membranes. Of note, the megaloblastic anemia resulting from folate deficiency is identical to that resulting from vitamin B12 deficiency, and therefore specific biomarker testing is essential to provide a differential diagnosis (See Section 22a.2). Causes of low and deficient folate status. Folate deficiency arises from various causes, relating either to increased requirements, reduced availability, or both. Pregnancy is a time when folate requirement is greatly increased in order to sustain the demand for rapid cell replication and growth of fetal, placental and maternal tissue (McNulty et al., 2019). Gastrointestinal conditions, such as celiac disease, can also lead to deficient folate status through chronic malabsorption. Certain drugs, including phenytoin and primidone (anticonvulsants) and sulfasalazine (used in inflammatory bowel disease), are also associated with folate deficiency through adversely affecting folate metabolism. Likewise, heavy alcohol consumption and smoking are also linked with lower folate status (Bailey et al., 2015). Prevalence of deficiency. Folate deficiency occurs commonly in pregnant and lactating women in low- and middle-income countries, where it is reported to occur in > 20% of women of reproductive age (Rogers et al., 2018). Here, dietary intakes of folate are often inadequate to meet the high requirements of pregnancy. In high-income countries, clinical folate deficiency is less common (due largely to the beneficial effects on folate status of supplementation and/or food fortification), but subclinical deficiency (indicated by low serum and RBC folate concentrations) is widespread, particularly in women of reproductive age, especially if pregnant and lactating, in European countries. Low folate status has also been reported in low birth weight and premature infants (Scholl & Johnson, 2000) and adolescents of low socioeconomic status (Bailey et al., 2015). Serum and RBC folate concentrations typically decrease throughout pregnancy; however, supplementation with folic acid prevents this decline and can thus prevent the occurrence of megaloblastic anemia of pregnancy (Blot et al., 1981; McNulty et al., 2013). Estimates of folate deficiency can however vary considerably across different populations and population sub-groups, depending on the method used and the cutoff points applied to biomarker measures of folate status (Bailey et al., 2015). Neural tube defects. Maternal folate nutrition before and in early pregnancy is known to play a critical role in fetal development. Notably, conclusive scientific evidence published 30y ago shows that folic acid supplementation with folic acid (the synthetic form of the vitamin) in early pregnancy protects against both first occurrence (Czeizel & Dudás, 1992) and recurrence (MRC, 1991) of neural tube defects (NTDs). These major birth defects occur as a result of a failure of the neural tube to close properly in the first few weeks of pregnancy, leading to death of the fetus or newborn, or to various disabilities involving the spinal cord, the most common form of which is spina bifida. The conclusive evidence that folic acid can prevent NTD has led to clear folic acid recommendations for women of reproductive age which are in place worldwide. It is important to appreciate, however, that the risk of NTD is increased when maternal folate status is low, but not necessarily within the range typically classed as folate deficiency. In a large prospective study of women in Ireland (where rates of NTD are among the highest in the world), a woman's risk of having a child with an NTD was found to be strongly associated with pregnancy RBC folate in a continuous dose-response relationship (Daly et al., 1995). In practice, implementing folic acid recommendations so that women and their babies benefit, is somewhat challenging. Although mandatory folic acid fortification of foods has proved to be highly effective in reducing NTD wherever it has been introduced (in 84 countries worldwide), elsewhere preventable NTDs are not being prevented including in European countries. Notably, one recent study estimated that from 1998 to 2017, a total of 95,213 NTD pregnancies have occurred amongst 104 million births in 28 European countries; a prevalence of 0.92 per 1,000 births (Morris et al., 2021). This study concluded that failure to implement mandatory folic acid fortification in the 28 European countries continues to cause NTD to occur in almost 1,000 pregnancies every year. The precise mechanism explaining the beneficial effects of periconceptional folic acid against NTD remains uncertain, though proposed mechanisms have focussed on factors that impair normal folate metabolism, including polymorphisms in folate genes. Among the latter, an increased risk of NTD is most strongly associated with the 677C>T variant in the gene encoding the folate-metabolising enzyme methylenetetrahydrofolate reductase (MTHFR), as reported in most studies and meta-analyses (Botto & Yang, 2000; Vollset & Botto, 2004). Autoantibodies against folate receptors are also implicated in pregnancies affected by NTD (Rothenberg et al., 2004). Also, although low maternal folate is considered the major contributing factor in NTD, convincing evidence shows that low vitamin B12 is an independent risk factor in NTD (Molloy et al., 2009). In addition, apart from preventing NTD, there is good evidence that periconceptional folic acid use may prevent congenital heart defects in infants (van Beynum et al., 2010), and possibly orofacial clefts (Bailey et al., 2015).22a.1.3 Food folate sources, bioavailability and dietary intakes
Folates are widely distributed in animal and plant foods, but only certain foods are rich sources, including liver, yeast, green leafy vegetables, asparagus, beans, legumes, where they exist primarily as polyglutamates, containing up to nine glutamate residues attached to the p‑aminobenzoic group of the molecule (Figure 22a.1). In contrast, folic acid, the synthetic vitamin, is a monoglutamate, containing only one glutamic acid residue in its structure. Also, unlike folic acid, which is a fully oxidized molecule, natural folates are reduced at the 5, 6, 7 and 8 positions of the pteridine ring and so are prone to oxidative cleavage at the C9‑N10 bond producing two degradation products, a pteridine and p‑aminobenzoylglutamate, both of which are inactive and cannot be biologically converted to any active folate form. Folate bioavailability. Bioavailability refers to the proportion of ingested nutrient that is absorbed and available for metabolic processes. Naturally-occurring food folates are only partially bioavailable (McNulty & Pentieva, 2004). Natural food folates are reduced molecules, and therefore are inherently unstable outside living cells. In addition, the ease with which folates are released from different food matrices and their conversion to the monoglutamate form before uptake by intestinal cells can vary greatly. Folate bioavailability from different food sources is also dependent on the presence of certain dietary constituents, that may enhance folate stability during digestion, or inhibit bioavailability owing to specific inhibitors of deconjugation. Thus, the bioavailability of food folates from a mixed diet is limited and highly variable. In addition, natural food folates (particularly green vegetables) can be unstable during cooking, and this can substantially reduce the folate content of a food before it is ingested (McKillop et al., 2002). This is an additional factor that further contributes to the limited potential for natural food folates to positively influence folate status (Cuskelly et al., 1996). Dietary folates. In contrast to the natural folate forms which have limited stability and bioavailability, folic acid provides a highly stable and bioavailable vitamin form. The bioavailability of folic acid is assumed to be 100% when ingested as a supplement, while folic acid in fortified food is estimated to have about 85% the bioavailability of supplemental folic acid (Pfeiffer et al., 1997). These differences have led to the development of “dietary folate equivalents” or DFE values; more details are given in Section 8a. Briefly, expressing dietary folate intakes and recommendations in DFE terms, allows an adjustment for the differences in bioavailability between natural food folates and the synthetic vitamin. The DFE is defined as the quantity of natural food folate plus 1.7 times the quantity of folic acid in the diet; this definition is based on the assumption that the bioavailability of folic acid added to food is greater than that of natural food folate by a factor of 1.7 (Institute of Medicine, 1998). This estimation is largely dependent on a metabolic study in non-pregnant women that estimated the bioavailability of food folates to be 50% relative to that of folic acid (Sauberlich et al., 1987), and other evidence, mentioned above, showing that folic acid added to food has 85% of the bioavailability of free folic acid (Pfeiffer et al., 1997). Fortified foods as a source of folate. Food fortification, the process of adding essential micronutrients to food, plays an important role in facilitating a more optimal nutritional status in individuals and populations. In the case of folate, it plays a crucial role. Folic acid, the folate form used for food fortification, is cheap to produce, very stable once added to foods and highly bioavailable when ingested. Thus, depending on national fortification policy and/or access to folic acid-fortified foods, the folate status of populations can vary greatly from one country to the next This is reflected in differences in health outcomes (notably in relation to NTD risk). Food fortification may be undertaken on a voluntary or mandatory basis. Voluntary fortification, whereby folic acid is added to foods such as breakfast cereals at the discretion of the manufacturer, is permitted in most European countries. This results in higher folate intake and status (Hopkins et al., 2015), but the benefit is limited only to consumers who choose to eat the fortified food products. However, when fortification is undertaken on a mandatory (population-wide) basis, including in the USA, Canada, Australia and Chile, it has proved to be highly effective, not only in increasing folate status and reducing folate deficiency (Yang et al., 2010), but also in reducing NTD in that country (Honein et al., 2001, Lopez-Camelo et al., 2005, De Wals et al., 2007, Sayed et al., 2008). Mandatory fortification is now in place in 85 countries worldwide, both high‑ and low-middle income countries Effects of high intakes of folate. High folate intakes are generally not associated with any adverse effects. However, there are concerns of potential adverse effects of excess intakes of folate acid, the synthetic vitamin form. Excessive folic acid intake constitutes exposure doses that exceed the Tolerable Upper Intake Level (UL) of 1000µg/d for adults, as set by the U.S. Institute of Medicine (1998). Historically the concern regarding excess folic acid focused on the potential to mask pernicious anemia and exacerbation of the clinical effects of vitamin B12 deficiency. More recently other concerns have been raised, including potential adverse effects on cancer risk, birth outcomes, and other diseases. However, a recent report from a 2019 expert workshop tasked with reviewing this research area, concluded that there is an insufficient body of evidence to support adverse human health outcomes as a result of high intakes of folic acid. Nonetheless, these experts called for further research to determine the safety of excess folic acid intake (Maruvada et al., 2020).22a.2 Biomarkers of folate status
In 2015, an expert international panel produced a comprehensive review of folate biology and biomarkers as part of the Biomarkers of Nutrition for Development (BOND) project convened by the U.S. National Institutes of Health (Bailey et al., 2015). This review identified serum folate, red blood cell (RBC) folate and plasma homocysteine as the “Priority Folate Biomarkers” for assessing folate status. Serum folate reflects recent dietary intake. RBC folate, compared with serum folate, is a better indicator of folate intake and status over the previous 3–4mos. Plasma homocysteine provides a functional indicator of folate status and is elevated in folate deficiency, on the basis that normal homocysteine metabolism requires an adequate supply of folate. Concentrations of folate in erythrocytes, but not serum, also fall in vitamin B12 deficiency. Ideally, both serum and RBC folate concentrations should be measured. Cut-off values for assessment of folate status. The sequential stages in the development of folate deficiency were originally established through detailed depletion / repletion experiments conducted during the late 1960's, providing a basis for setting cut-off values for serum and RBC folate for the assessment of status (Herbert, 1987); these sequential stages of folate deficiency and related cut-off values are summarized in Box 22a.1Box 22a.1. Cut-off values for assessment of folate status in populations
Historical perspective
During the late 1960’s, cut-off values for sequential stages of folate deficiency were established through depletion/repletion experiments
(Herbert, 1987):
The initial stage of folate deficiency, termed negative folate
balance, is consistent with serum folate values of < 7nmol/L
(Herbert, 1987).
If the negative balance persists, tissue folate
becomes depleted, as indicated by RBC folate falling below the
normal range to < 363nmol/L. At this second stage, there is little
evidence that biochemical function is impaired, although plasma
homocysteine concentrations may be slightly elevated. By the
third stage, termed folate-deficient erythropoiesis, functional
impairment is usually evident, with erythrocyte folate values
< 272nmol/L. Tissue folate stores are severely depleted in the
fourth and final stage where the classical hematological changes
occur, manifested as folate-deficiency anemia. These include
macro-ovalocytic erythrocytes in the circulating blood and
megaloblasts in the bone marrow. At this stage, hypersegmented
neutrophils in the peripheral blood smear and abnormal RBC
indices are also apparent; mean red cell volume and mean cell
hemoglobin are elevated and hemoglobin concentration is low.
More recently, revised cut-off points for
folate deficiency (serum folate <10nmol/L and
RBC folate < 340nmol/L) have
been defined based on the metabolic indicator,
plasma homocysteine
(Selhub et al., 2008),
as summarized in Box 22a.1.
- A serum folate value < 7nmol/L (3ng/mL) indicated negative folate balance
-
For RBC folate, cut-off values were set at:
- < 363nmol/L (160ng/mL) to indicate the onset of folate depletion,
- < 272nmol/L (120ng/mL) to mark the beginning of folate-deficient erythropoiesis, and
- < 227nmol/L (100ng/mL) to indicate folate-deficient anemia
- Serum folate < 10nmol/L to indicate folate deficiency
- RBC folate < 340nmol/L to indicate folate deficiency
22a.2.1 Serum and RBC folate concentrations
Serum folate represents the sum of several folate forms circulating in blood. The main circulating folate is 5‑methyl‑THF; other reduced forms such as THF and formyl‑THF may also be present, but in very small concentrations (Bailey et al., 2015). As will be discussed later (section 22a.2.4), unmetabolized folic acid can also be present in varying concentrations in plasma (Bailey et al., 2010). Red cells contain much higher folate concentrations than serum. 5‑methyl‑THF polyglutamates are the main RBC folate forms. The measurement of RBC folate is more complex than that of serum folate, because of the need to convert polyglutamates to monoglutamates prior to analysis. In individuals homozygous for the MTHFR C677>T polymorphism, a portion of the 5‑methyl‑THF polyglutamates is replaced by formyl-folates (Bagley & Selhub, 1998). Serum folate is the earliest indicator of altered folate exposure and reflects recent dietary intake (Pfeiffer et al 2010). In the individual, serum folate concentrations can increase markedly in response to dietary folate, reaching a peak concentration 90min after ingestion. RBC folate is a sensitive indicator of long-term folate status. RBC folate parallels liver concentrations, accounting for about 50% of total body folate, and thus reflects tissue folate stores (Wu et al., 1975). RBC folate, compared with serum folate, responds more slowly to changes in dietary folate intake and is a better indicator of folate intake over the previous 3–4mos when circulating folate is incorporated into the maturating red cells during erythropoiesis, thereby reflecting folate status during the preceding 120d, the half‑life of the RBC (Mason, 2003). Factors affecting serum and RBC folate concentrations Dietary folate intakes. Blood folate levels are primarily affected by dietary folate intakes. Serum and RBC folate are highly responsive to intervention with folic acid (Duffy et al., 2014), with natural food folates typically resulting in poorer folate responses compared to folic acid at similar intervention levels (Cuskelly et al., 1996). Likewise, population data show that both serum and RBC folate concentrations are highly reflective of exposure to folic acid, with the highest concentrations observed in people who consume folic acid in both supplements and fortified foods (Yang et al., 2010; Hopkins et al., 2015). Vitamin B12 status. Because vitamin B12 is required for normal folate recycling and folate retention within cells, vitamin B12 deficiency leads to a failure to retain folate within cells (Hoffbrand & Weir, 2001). Consequently, RBC folate concentrations fall, despite the presence of normal (or sometimes even elevated) serum folate. Thus, low RBC folate may reflect vitamin B12 deficiency as well as folate deficiency. Fasting versus non fasting samples. Samples from fasted individuals, on average, have lower concentrations of serum (by 10%) and RBC folate (by 5%) compared with samples from nonfasted (< 3h) participants, but the small differences generally indicate that fasting is not essential when assessing the folate status of populations. (Bailey et al., 2015). Important preanalytical factors. Detailed information on preanalytical factors affecting serum and RBC folate is described in a comprehensive review article on analytical approaches by Pfeiffer et al.,2010. Folate is the least stable of the B vitamins and is susceptible to oxidative degradation during preanalytical sample handling and storage. Careful sample handling and use of antioxidants are therefore required to maintain sample integrity. Blood for serum folate analysis should be processed and frozen promptly. Although serum is generally preferred over plasma in most laboratories, both matrices generally produce comparable results for folate, as long as the sample processing is not delayed. If delays are unavoidable, the sample should be protected from light, kept cool and processed within a few days of collection. Folate in serum and hemolysates (but not in whole blood) can withstand a few short freeze / thawing cycles, particularly if the vials are kept closed as much as possible to minimize the exposure of the sample to air. Folate in serum / plasma degrades rapidly at room temperature, particularly in the presence of EDTA. Analytical methodologies for measurement of serum and RBC folate Over the past 50y, analytical methods to assess serum and RBC folate concentrations have been continuously improved; however, they have not yet reached the point where they produce sufficiently comparable results across methods or laboratories. The within-person variability for serum folate is about twice that for RBC folate (CV of 21.5% and 9.1%, respectively, Bailey et al., 2015). Critical considerations in folate assessment. Assessing folate status is complicated by the large number of folate forms that may be readily interconverted. To overcome this problem, microbiological assays have been used for many decades due to the ability of some bacteria to grow in the presence of many different forms of folate, i.e. L.rhamnosus responds to all active monoglutamate forms (see below). Subsequently, assays using competitive protein binding became common because of their simplicity. In more recent years, the use of LC‑MS/MS has emerged. Of note, the emergence of LC‑MS/MS enables investigation of relevant research findings related to folate metabolism. These include alterations in the relative proportion of different folate forms in red cells owing to the common MTHFR C677>T polymorphism, along with an overall lower RBC folate (Molloy et al., 1997; Bagley & Selhub, 1998; Shane et al., 2011), and the presence of free (“unmetabolized”) folic acid in the blood as a result of high intakes of folic acid (Pfeiffer et al, 2010). There are three main method types for measurement of serum and RBC folate, each with advantages and disadvantages (Bailey et al., 2015). Microbiological assay. This is widely considered to be the gold standard assay for serum and RBC total folate because it measures all biologically active forms equally and does not measure folate species that lack vitamin activity. The underlying principle of the microbiological assay is that a folate-dependent microorganism, namely Lactobacillus rhamnosus (formerly called Lactobacillus casei), grows proportionally to the amount of folate present in serum or whole blood and the folate concentration can be quantified by measuring the turbidity of the inoculated medium after a 2d incubation. A chloramphenicol-resistant strain of L.rhamnosus is used and the assay is performed using automated microtiter plate technology. The key advantages of the microbiological assay include its excellent sensitivity, low cost and simple instrumentation (thus suited for low-resource settings), and the fact that it can be used with dried blood spot samples. The high sensitivity is a particular advantage when limited volume is available, such as for samples collected from a fingerstick or dried blood spot. The disadvantages are that the microbiological assay is relatively laborious unless automated liquid handling is introduced, lengthy assay time with limited throughput and limited linear range (thus requiring dilution of samples). It is also prone to contamination issues and potential interference by the presence of antibiotics or antifolates. The latter limitation is well recognized, but in practice may not be such an issue, in that analysts in the US reported that < 1% of samples from the population-based NHANES cohort exhibited a pattern of interference due to the presence of antibiotics or antifolates (Pfeiffer et al, 2010). Protein-binding assays. These assays were developed primarily for clinical settings, to enable the diagnosis of folate deficiency. Protein-binding assays use the highly specific folate-binding protein to extract folate from the sample. The strengths of this approach include the high sample throughput, quick turnaround time, availability in commercial kit form and minimum operator involvement. The disadvantages are that the various folate forms have different affinities to folate-binding protein, the questionable accuracy when mixtures of folate are present, the limited linear range (thus requiring dilution of samples), matrix effects when sample is diluted, and lot-to-lot variability of commercial kits. Also, although not sensitive to antibiotics, protein-binding assays are influenced by certain antifolates such as methotrexate. Chromatography-based assays (HPLC-FD, LC‑MS/MS). Chromatography-based methods typically provide information on individual folate forms based on measurement of intact folates via HPLC. More recently, LC-tandem mass spectrometry (LC‑MS/MS) is now the preferred detection for HPLC-based methods in specialized laboratories. Advantages of chromatography-based approaches are that they measure all folate forms, are highly selective and specific, have good sensitivity and precision, enable in-house control of performance and use of stable-isotope-labeled internal standards to compensate for procedural losses. Ensuring accurate calibration is a big task for chromatography-based methods, due to the high number of folate forms and also because of their instability. Disadvantages include the high costs and requirement for expensive instrumentation, experienced operator and frequent technical service, along with being a relatively laborious approach unless automated liquid handling is introduced, and the requirement for complex sample extraction / cleanup. Also interconversions of folate forms during the assay procedure need to be considered in interpretation of data. Cut-off values and Interpretation of Serum and RBC folate The measurement of total folate provides information on the folate status of the individual, either in the short-term through serum folate, or in the long-term through RBC folate. An historical perspective on the use of folate cutoffs is provided in Box 22a.1. However, the inconsistent use of cutoff values over time to assess the proportion of populations with deficient or low folate status led to a certain degree of confusion. More recently, revised cut-off points for folate deficiency (serum folate < 10nmol/L and RBC folate < 340nmol/L) have been defined based on the metabolic indicator, plasma homocysteine (Selhub et al., 2008), as summarized in Box 22a.1. These cutoff values have been recommended by the WHO for assessing folate status of populations (de Benoist, 2008). Of note, the values were derived from data generated using the microbiological assay. As discussed earlier, the risk of NTD is associated with a maternal folate status that would not conventionally be classed as deficient. Although there is no cutoff value established by international organizations for folate concentrations to define NTD risk in populations, it is accepted that the number of NTDs that can be prevented in a population is dependent on maternal folate status. Specifically, RBC folate concentration in the mother has been shown to be a sensitive biomarker of NTD risk. In the one and only prospective study that has been conducted to date, Daly et al. (1995) found that the prevalence of NTD in an Irish population was lowest when maternal RBC folate concentrations were ≥ 906nmol/L (400ng/mL). Subsequently, data modeled from folic acid intervention studies in China by Crider et al. (2014) confirmed the dose response relationship between RBC folate concentrations and NTD risk as shown in the Irish study (Daly et al., 1995).22a.2.2 Plasma homocysteine concentrations
Plasma homocysteine provides a sensitive functional indicator of folate status. On the basis that normal homocysteine metabolism requires an adequate supply of folate, plasma homocysteine becomes elevated when folate status is low. Homocysteine is a four-carbon, thiol-containing amino acid found in human plasma, mostly present in the form of various disulfides, such as homocysteine-cysteine disulfide. About 75% of total homocysteine is bound to protein (mainly albumin), whereas the remainder occurs in nonprotein-bound “free” forms. Total homocysteine is defined as the sum of all homocysteine species in serum or plasma, including free and protein-bound forms. Only a very small portion (1–2%) of plasma homocysteine is present as the thiol, however the relative contribution of the thiol to total homocysteine increases to 10–25% in patients with abnormally elevated plasma homocysteine (Bailey et al., 2015). Homocysteine is derived from the essential amino acid, methionine. It is metabolized in two ways: remethylation to methionine (by methionine synthase) or transsulfuration to cystathionine (by cystathionine beta-synthase; CBS) then to cysteine (Figure 22a.2). These pathways require adequate status of folate and the metabolically related B‑vitamins. The remethylation pathway, whereby methionine is synthesized from homocysteine by methionine synthase, is dependent on both folate and vitamin B12 as cofactors, whilst the transsulfuration pathway that converts homocysteine to cysteine is catalyzed by two vitamin B6 dependent enzymes. A fourth B vitamin, riboflavin, is required in its cofactor form flavin adenine dinucleotide (FAD) for the activity of MTHFR, the enzyme that catalyzes the reduction of 5,10 methyleneTHF to 5 methylTHF. Once formed, 5 methylTHF is used by methionine synthase for the vitamin B12-dependent conversion of homocysteine to methionine and the formation of THF. Thus, the concentration of homocysteine in plasma / serum is regulated by up to four B‑vitamins: folate, vitamin B12, vitamin B6 and riboflavin. Apart from providing a functional biomarker of folate, higher plasma homocysteine is associated with a number of chronic diseases of ageing, including an increased risk of cardiovascular disease (CVD; Graham et al., 1997), cognitive impairment and dementia (Smith & Refsum, 2016). It remains to be established, however, whether plasma homocysteine per se a risk factor for these or other diseases. In the case of CVD, this issue is particularly controversial. Despite strong and consistent evidence from observational studies over many years, several secondary prevention trials published between 2004 and 2014 failed to demonstrate a benefit of homocysteine-lowering therapy against the recurrence of CVD events in patients with existing disease. The evidence is however stronger for the relationship of homocysteine with stroke than heart disease, with good evidence from both population data and randomized trials that folic acid intervention and/or homocysteine-lowering can significantly reduce the risk of stroke, and particularly so in people with no previous history of stroke (McNulty et al., 2017). Of note, although the literature in this area focuses on homocysteine as the CVD risk factor, it is possible that folate and related B‑vitamins have roles in CVD that are independent of their homocysteine-lowering effects. Thus, plasma homocysteine may be linked with CVD as a functional marker of low B‑vitamin status which reliably reflects perturbed one-carbon metabolism, rather than being causatively related to CVD per se (McNulty et al., 2017). Factors affecting homocysteine concentrations Folate status. On the basis that normal homocysteine metabolism requires an adequate supply of folate, plasma homocysteine is first and foremost affected by folate status. Thus, plasma homocysteine was shown to respond within 3–4wks of folate depletion (increase) and subsequent repletion (decrease) in a controlled metabolic study in healthy women (Jacob et al., 1998). Likewise, in observational studies, plasma homocysteine is invariably found to be inversely associated with folate status, whether measured as serum or RBC folate. Intervention with folic acid. Plasma homocysteine is highly responsive to intervention with folic acid (the synthetic vitamin form), alone or in combination with vitamin B12, vitamin B6, riboflavin and betaine (or choline). Thus, food fortification with folic acid has marked effects on homocysteine concentrations. Using population-based data from the US, Pfeiffer et al. (2008) reported a 10% decrease in plasma homocysteine when comparing values pre-fortification (1991–1994) to post-fortification (1999–2004) of food with folic acid, as implemented on a mandatory basis in 1996–1998. Folic acid-fortification on a voluntary basis (i.e. added to food at the manufacturer's discretion) also affects plasma homocysteine. In a convenience sample of nearly 500 healthy adults in Northern Ireland aged 18–92y, who were not taking folic acid supplements, homocysteine concentrations were lower by 2µmol/L in high consumers compared to non-consumers of fortified foods (providing > 100µg/d and 0µg/d folic acid, respectively) (Hoey et al., 2007). Folate-related B vitamins. Because normal homocysteine metabolism is dependent on four B vitamins, homocysteine concentrations will be elevated with other B vitamin deficiencies apart from folate, notably vitamin B12 (Allen et al., 2018). Thus whilst plasma homocysteine is primarily a folate biomarker, once folate status is optimized, a much greater dependency on vitamin B12 emerges (Quinlivan et al., 2002). Likewise, in population groups who consume folic acid-fortified foods or folic acid supplements, homocysteine is considered to be a more reliable biomarker of vitamin B12 than folate (Refsum et al., 2004). Disease and lifestyle. Apart from inversely reflecting folate and related B vitamin status, plasma homocysteine is found to be elevated in patients with impaired renal function (Yetley et al., 2011; Allen et al., 2018) and with certain drug treatments (Refsum et al., 2004). Alcohol intake, coffee-drinking and smoking are also associated with higher plasma homocysteine. Age, sex and lifecycle stage. Homocysteine concentrations increase throughout life and are higher in males compared to females at all ages. Although plasma homocysteine decreases in pregnancy (by about 50%), concentrations are reported to normalize within a few days postpartum (Murphy et al., 2004). Ethnic and genetic effects. Plasma homocysteine may also differ among ethnic groups, but these differences appear to be less important than the influence of B vitamin status. Notably, the most common genetic cause of elevated homocysteine in the general population is homozygosity for the MTHFR C677>T polymorphism (affecting an estimated 10% of people worldwide but much higher in some populations, including Mexico and Northern China). This folate gene variant also contributes to a higher risk of blood pressure throughout the lifecycle, particularly when combined with deficient status of riboflavin, which is required as a cofactor for MTHFR, a key folate metabolizing enzyme (Psara et al., 2020; Ward et al., 2020). Notably, both phenotypes associated with this common polymorphism (high homocysteine and high blood pressure) appear to be modifiable with better riboflavin status (McNulty et al., 2020). Most other genetic polymorphisms in enzymes related to one-carbon metabolism have little effect on homocysteine concentrations (Refsum et al., 2004). Inborn errors of metabolism. Homocystinuria refers to rare inborn errors of metabolism which lead to severely increased homocysteine concentrations in plasma, usually > 100µmol/L, along with large amounts excreted in urine. The most common cause of homocystinuria is deficiency of the enzyme cystathionine β‑synthase (CBS). CBS deficiency, an autosomal recessive condition, has a reported worldwide birth prevalence of 1 in 344,000, while that in Ireland the frequency is much higher, at 1 in 65,000, based on newborn screening and cases detected clinically. These patients have a high risk of premature, frequently fatal, thromboembolic events. Early diagnosis and treatment with pyridoxine and/or folic acid and betaine, preferably from infancy, can however prevent CVD events and most of the clinical symptoms (Refsum et al., 2004). Analytical methodologies for measurement of plasma homocysteine Plasma total homocysteine is a very stable analyte as long as the plasma is separated from the red blood cells within 1hr of blood collection (or within 8 hours if the whole blood is kept on ice). As described in detail by Refsum et al. (2004), various method types are available for homocysteine determination. These range from fully automated commercial kits (immunoassay or enzymatic methods) to chromatographic assays with mass spectrometry detection, overall providing comparable results and good assay performance. All methods require the reduction of the disulfide bonds to allow measurement of total homocysteine. The reported within-person variability CV for plasma homocysteine is 12.2% (Refsum et al., 2004). Choice of method. Because the measurement of plasma homocysteine produces comparable results across different method types, the choice of method is mainly dependent on available instrumentation and technical expertise. Bailey et al. (2015) put forward the following recommendations to help in making this decision:- Using commercial kits (either immunoassay or enzymatic assay) on a fully-automated clinical analyzer will provide the highest throughput and quickest turnaround time with the least effort. The main disadvantage is the relatively high reagent costs, which can make the measurements quite expensive, particularly for a large number of samples.
- If an HPLC system with fluorescence detector is available, setting up a manual assay may be the least expensive approach, particularly in settings with low labor costs. The disadvantage of the manual HPLC assay is that the number of samples that can be run is quite limited (typically to about 50–70 unknown samples per analytical run).
- If a laboratory has access to an existing LC‑MS/MS system and the required technical knowhow, plasma homocysteine can be measured in a high throughput semi-automated manner, with quite low reagent costs. Due to the high initial cost, however, an LC‑MS/MS system is economical only if a large number of samples are measured regularly.
- GC-MS or GC-MS/MS have also been used in high throughput research settings, particularly when the co-determination of methylmalonic acid and methionine is of interest.
- GC-based methods provide better precision, higher resolution, and longer column life compared to LC-based methods and are not subject to ion suppression issues, which can be a problem in LC‑MS/MS methods.
Population sub-group | Folic acid Supplemented1 |
Non- supplemented |
---|---|---|
Pregnancy | > 8 | > 10 |
Children < 15y | > 8 | > 10 |
Adults 15–65y | > 12 | > 15 |
Adults > 65y | > 16 | > 20 |
- Each laboratory should establish reference limits for its region.
- The reference population should exclude individuals with folate or cobalamin deficiency or increased creatinine, and patients with diseases or who are taking drugs that increase homocysteine concentrations.
- Separate reference limits for children, adults, the elderly, and pregnant women should be used (Table 22a.1).
- In populations consuming folic acid supplements or folic acid-fortified foods, the upper reference limit is usually 20–25% lower than in nonfortified populations (Table 22a.1).
- The upper homocysteine reference limit should be interpreted with age, sex, and other determinants in mind, particularly prevailing food fortification practices.
22a.2.3 Other biomarkers of folate status
Neutrophil lobe count Usually, neutrophils have three or four segments, but in megaloblastic anemia (owing to folate or vitamin B12 deficiency), this number increases. Neutrophil hypersegmentation can be evaluated in smears of peripheral blood or in white blood cells obtained from the buffy coat (i.e. interface between the serum / plasma and the sedimented red cells), and may be the earliest morphological change to appear in the blood in folate and B12 deficiency. Neutrophil hypersegmentation however is not specific to folate or B12 deficiency as it also occurs in other conditions including uremia, myeloproliferative disorders, myelofibrosis and as a congenital lesion in approximately 1% of the population, even when the status of folate and vitamin B12 is adequate. The observed greater incidence of neutrophil hypersegmentation in iron deficiency anemia concurrent with folate and/or vitamin B12 deficiency implies interactive effects on the erythropoietic process (Westerman et al., 1999; Metz, 2008). Serum folic acid Unmetabolized (or “free”) folic acid can be present in varying concentrations in plasma and is typically associated with higher folic acid intake via fortified foods, supplements, or a combination of both (Bailey et al., 2010). Whilst greater concentrations of unmetabolized folic acid are generally associated with higher serum folate concentrations, there is large variation in reported values and no clear dose response relationship exists between folic acid intake and unmetabolized folic acid in plasma (Pfeiffer et al., 2004; Bailey et al., 2010; Obeid et al., 2011). Thus, cut-off values or desirable ranges have yet to be identified. One randomized trial addressed concerns relating to the potential for folic acid to exert adverse biological effects in pregnancy, and demonstrated that folic acid supplements taken at recommended levels throughout pregnancy do not lead to increased circulating unmetabolized folic acid in mothers or their babies (Pentieva et al., 2016). However, the biological impacts and the significance for health in general of unmetabolized folic acid in blood remain to be elucidated. Genomic biomarkers of folate status DNA cytosine methylation. Apart from its role in DNA synthesis, folate plays an important role in DNA metabolism as it is required for the synthesis of methionine and thus SAM, which in turn is required as a methyl donor for the maintenance of cytosine methylation, essential for silencing of genes or structural integrity of specific regions of chromosomes (Fenech, 2012). When SAM is depleted, the maintenance of DNA methylation may become compromised, leading to hypomethylation of cytosine and structural changes in chromatin. Some studies suggest that global DNA methylation status is reduced when folate is deficient (Kim, 2005; Crider et al., 2012), but findings in this regard are somewhat inconsistent. Uracil misincorporation into DNA. Measurement of uracil content in DNA may also provide a biomarker of folate status, on the basis that adequate amounts of folate as 5,10‑methyleneTHF are required by thymidylate synthase to convert deoxyuridine monophosphate (dUMP) to thymidylate monophosphate (dTMP) in pyrimidine synthesis and thus DNA biosynthesis. If 5,10‑methyleneTHF is limiting as a substrate of thymidylate synthase, dUMP accumulates and it becomes more probable that uracil is incorporated into DNA instead of thymidine during DNA synthesis (Stover, 2009; Fenech, 2012). Micronuclei. Micronuclei have the same morphological features as normal nuclei but are much smaller. Excessive uracil incorporation into DNA and hypomethylation of DNA can lead to the formation of micronuclei from chromosome fragments which can be measured in lymphocytes or in erythrocytes (Blount et al., 1997; Fenech, 2012). Observational studies show that micronuclei in lymphocytes or erythrocytes are inversely associated with dietary folate intake and RBC folate (Blount et al., 1997), whilst intervention studies have reported a significant reduction in micronuclei frequency in lymphocytes with folic acid supplementation (Fenech et al., 1997). It is now possible to score micronuclei automatically and reliably using a wide range of image cytometry platforms making this technique amenable to mass screening. Given its sensitivity to folate deficiency, micronuclei measurement, in combination with uracil and DNA methylation measurements, can potentially provide a reliable assessment of genome pathology resulting from deficient folate status (Bailey et al., 2015).22a.2.4 Assessment of folate status in children
Most studies assessing folate status have focused on adults. Far fewer studies provide folate biomarker data for children, with notable exceptions being published reports using data from population-based surveys conducted in the United States (Pfeiffer et al., 2012) and the United Kingdom (Kerr et al., 2009;. Figure 22a.3) The latter report proposes normal ranges for folate biomarkers for use in clinical pediatric settings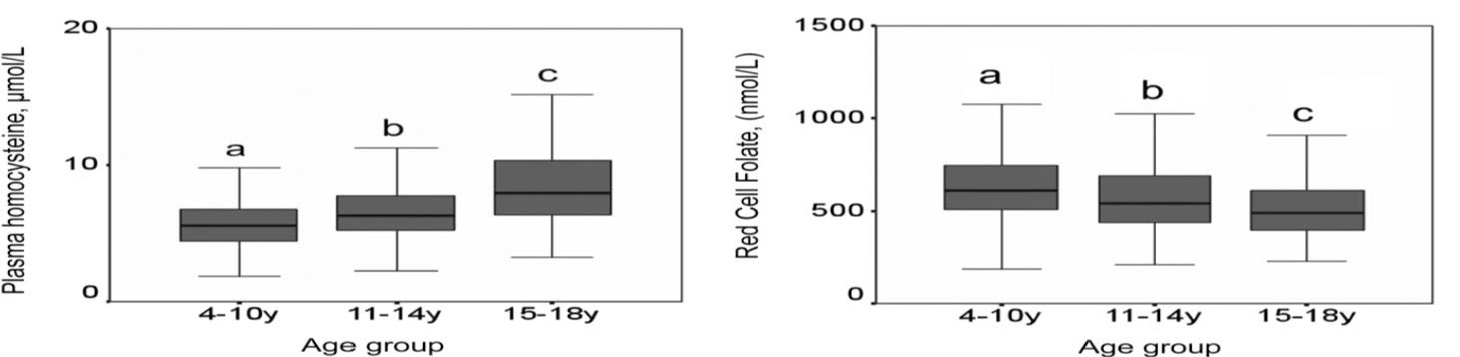