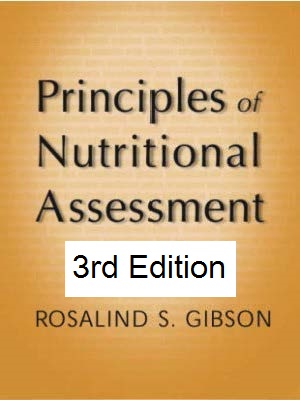
Gibson RS1,
Principles of
Nutritional Assessment:
Body Composition:
Laboratory Methods
3rd Edition, July 2024
Abstract
Several indirect in vivo laboratory methods are available to assess body composition. Their selection depends on the study objective, the precision and accuracy required, the cost, convenience to the subject and their health, and equipment and technical expertise available. This chapter describes both non-scanning and scanning in vivo laboratory techniques. Non-scanning techniques include total body potassium, total body water (via isotope dilution or bioelectrical impedance analysis (BIA)), neutron activation analysis, densitometry (via under-water weighing or air-displacement plethysmography), and total body electrical conductivity. Four scanning techniques are included: computerized tomography, magnetic resonance imaging, dual energy X‑ray absorptiometry (DXA), and ultrasound. Each technique generates body composition data in a different way, so the results are not interchangeable. For each technique, the characteristics, including both the advantages, limitations and assumptions applied to generate the body composition data, are outlined. In addition, their potential applications are summarized, with emphasis on the alterations in the relative proportions of the body components that may occur in certain life-stage groups or disease states. Such alterations may invalidate the determination of fat and fat-free mass in a 2‑component model of body composition. In a 2‑component model, constants for the hydration and density of fat-free mass which ignore the inter-individual variability in these properties, are often assumed.Many factors have the potential to alter values for these assumed constants, especially growth and maturation in children, aging, pregnancy, and obesity. Consequently, researchers have developed specific constants for the hydration and density of fat-free mass specific for these circumstances. Their use will improve the estimates of fat-free mass when applying the simple 2‑component model based on measurements of total body potassium, total body water, or whole-body density. From a comparison of nine in vivo body composition methods conducted by Field and co-workers (2015), measurement of densitometry via air displacement plethysmography was selected as the method with the highest degree of accuracy and reliability and with the least degree of technical error to track and monitor whole-body composition across the lifespan, provided any alterations in the relative proportions of the body components, are taken into account.
In clinical patients with certain disease states, over- or underhydration and abnormalities in mineral mass may occur, resulting in substantial variability in both the hydration and density of fat-free mass. In these circumstances, application of multi-component models that include measurements of protein and/or bone minerals (via neutron activation or DXA) as well as total body water and density will minimize assumptions related to the structure, hydration, and density of fat-free mass. The 4-component model is considered the criterion method whereby whole-body composition can be most accurately assessed. A simplified version based on measurements from both DXA and BIA holds promise for monitoring conditions in certain disease states.
The four scanning techniques are widely used in clinical settings to quantify in vivo body composition, especially at the tissue-organ level, when investigations of bone density, skeletal muscle mass, and the deposition of visceral ectopic fat with their corresponding relationship with osteoporosis, sarcopenia, or cardiometabolic risk, respectively, are needed.
CITE AS:
Gibson RS. Principles of Nutritional Assessment.
Body Composition: Laboratory Mehods.
https://nutritionalassessment.org/bodylabmethods/
Email: Rosalind.Gibson@Otago.AC.NZ
Licensed under CC-BY-4.0
( PDF ).
14.0 An introduction to techniques used to measure body composition
Accurate methods for measuring body composition are required in investigations of obesity, malnutrition, weight loss following bariatric surgery, muscle wasting, sarcopenia, osteopenia, and osteoporosis. Body composition information is also used to establish the appropriate prognosis and treatment of hospital patients, and with longitudinal assessment, to monitor the effects of interventions on body composition (Lemos & Gallagher, 2017). Selection of the method to measure body composition depends on the required precision and accuracy, the study objective, cost, convenience to the subject, their health, and equipment and technical expertise available (Lukaski, 1987). Methods based on multi-component models that include analysis of protein and minerals, minimize assumptions related to tissue density, hydration, and structure. This is important because in malnourished individuals, the elderly, and subjects with metabolic disturbances, the relative proportions of the body components is often altered, and losses of protein, fat, and bone mineral content may occur, often in association with the rapid accumulation of water. Changes such as these invalidate the determination of fat and the fat-free mass in the 2‑component model. Absolute validity cannot be assessed for any of the indirect in vivo body composition methods because the gold standard for body composition analyses is cadaver analysis. Instead, only relative validity can be assessed, defined as the comparison for each subject of the results from the “test” method with the results from another method, termed the “reference or criterion” method; the latter having a greater degree of demonstrated validity. A 4‑component model is now considered sufficiently accurate to act as a reference or criterion method, but its use in many settings is limited because of the expensive and sophisticated technology required. Multiple statistical approaches can be used to establish the validity of the “test” method compared with a reference method. They include regression and correlation analyses, paired t tests, and more recently, Bland-Altman analysis; see Earthman (2015) for further details. The characteristics of the various procedures used for measuring body composition are summarized in Box 14.1. This list includes both non-scanning and scanning techniques. Detailed sections (14.2‑14.12) describe each of these indirect in vivo methods now available to assess body composition. Comments on these methods are also given, along with the assumptions used, and the advantages and disadvantages of each method. Scanning techniques such as computer tomography (Section 14.9), magnetic resonance imaging (Section 14.10) and whole body dual energy X‑ray absorptiometry (DXA) (Section 14.11) are included together with a discussion of their clinical importance (Lee et al., 2019; Neeland et al., 2019). Each technique generates body composition data in different ways, so the methods are not interchangeable. Methods with the lowest cost are often the most imprecise. Methods employing the 2‑component model (i.e., body fat and fat-free mass) include total body potassium, total body water via isotope dilution or bioelectrical impedance, densitometry via hydrostatic weighing or air-displacement plethysmography, and total body electrical conductivity. Such methods are not suitable for clinical populations when the basic assumptions of the 2‑component model are often invalid. Instead, in these populations, techniques using a 3, 4, or 5‑component model should be applied. For example, (Section 14.11) has the capacity to generate data that can be used with a 3‑component model. See Lohman (1986) and Pietrobelli et al. (1996) for further details. Three scanning techniques — computerized tomography (Section 14.9), magnetic resonance imaging (Section 14.10), and DXA (Section 14.11) — can be used to quantify components (e.g., skeletal muscle, bone, visceral ectopic fat) at the tissue-organ level of body composition as well as to assess the relative proportions of the fat-free mass, body fat, and bone mineral content. Of these, only DXA has been recommended for the assessment of fat mass in patients with a variety of disease states; the use of DXA for the assessment of fat-free mass is not recommended for clinical populations because its validity for assessment of fat-free mass in any clinical population remains unknown (Sheean et al., 2020).- 14.1 Chemical analysis of cadavers
Cadaver analysis provides the gold standard data on body composition, but the use of such data is limited by ethical barriers. Results from a few older studies (1945‑1968) are mostly based on adults who had died because of illness. - 14.2 Total body potassium (TBK)
TBK is measured by counting radiation from naturally occurring 40K in a whole body counter. Required equipment is only found in specialized facilities. Estimates of the fat-free mass can be derived from the TBK. - 14.3 Total body water
from isotope dilution (TBW)
A tracer dose of water, usually labeled with the stable isotope 2H, is given orally or intravenously and then allowed to equilibrate. The concentration of the isotope in serum, urine, or saliva is measured and TBW calculated from dilution observed following equilibration. Obesity, pregnancy, and wasting disease increase TBW. - 14.4 Multiple dilution methods
Typically, multiple dilution involves determining both total body water via isotope dilution and extracellular water (ECW), the latter using a tracer such as bromide that does not enter the intracellular space. The difference between these two measurements (i.e., TBW − ECW) reflects the intracellular water. - 14.5 In vivo activation analysis
Radioactive isotopes of N, P, Na, Cl, Ca are created by irradiating the subject. The resulting γ‑radiation is measured using a whole body counter. Subjects are exposed to radioactivity. Sensitivity varies with the element. Required equipment is only found in specialized facilities. - 14.6 Densitometry
Body density is derived from measurements of body mass and body volume. The latter is calculated from: (a) the apparent loss of weight when the body is totally submerged in water — difficult with young children, the elderly or sick patients — or, (b) air-displacement or water-displacement plethysmography. - 14.7 Total body
electrical conductivity (TOBEC)
Subject lies supine in a solenoid coil through which a 5MHz current is passed. The conductivity value of the subject is obtained by subtracting the background value when the coil is empty. Edema, ascites, dehydration, electrolyte balance and variations in bone mass all interfere with the conductivity reading. - 14.8 Bioelectrical impedance (BIA)
The impedance to a weak electrical current passed between the right ankle and right wrist of a subject in supine position is measured. Edema, ascites, and dehydration invalidate single frequency measurements. Multifrequency measurements allow estimation of both total and extracellular compartments. - 14.9 Computerized tomography (CT)
Method measures attenuation of X-rays as they pass through tissues, the degree of attenuation being related to differences in physical density of the tissues. An image is reconstructed from the matrix of picture elements. Exposure to ionizing radiation limits use of CT for pregnant women or children. Expensive equipment. - 14.10 Magnetic resonance
imaging (MRI)
Imaging involves placing a subject in a very strong magnetic field and observing the relative differences in behavior of 1H protons in lean and adipose tissue. No exposure to ionizing radiation but equipment bulky and expensive. - 14.11 Dual
energy X‑ray absorptiometry (DXA)
Utilizes the attenuation of a dual energy X‑ray beam, often during whole-body scanning. New fan-beam technologies replacing earlier pencil-beam techniques lead to lower X‑ray doses and improved spatial resolution. High precision method, but results are calibration dependent and differences between various equipment manufacturers can be significant. - 14.12 Ultrasound
High-frequency sound waves from a combined ultrasound source and meter pass through adipose tissue to the adipose-muscle tissue interface. At the interface, some sound waves are reflected back as echoes, which are translated into depth readings via a transducer. CT, MRI, or DXA provides higher degree of structure resolution than ultrasound.
14.1 Chemical analysis of cadavers
Studies of body composition by direct chemical analysis of human cadavers are limited. Most of the cadavers were analyzed between 1945 and 1968 and were adults of varying ages who had died because of illness; hence, the values obtained may not be representative of an average healthy adult.Sex (y) | Water (g/kg) | Protein (g/kg) | Density (kg/m3 | Potassium (mmol/kg) |
---|---|---|---|---|
Male (25) | 728 | 195 | 1120 | 71.5 |
Male (35) | 775 | 165 | 1083 | − |
Female (42) | 733 | 192 | 1103 | 73.0 |
Male (46) | 674 | 234 | 1131 | 66.5 |
Male (48) | 730 | 206 | 1099 | − |
Male (60) | 704 | 238 | 1104 | 66.6 |
Mean | 724 | 205 | 1106 | 69.4 |
SD | 34 | 28 | 17 | 3.3 |
14.1.1 Applications of cadaver use
Table 14.1 presents data on the contribution of water and protein to the fat-free mass of six adult cadavers. The fat-free tissues of the cadavers were of a relatively constant composition, containing about 72% water and about 20% protein; the potassium content was also relatively constant (about 69mmol/kg). In contrast, the amount of fat was very variable (data not shown), ranging from 4.3% to 27.9% of body weight in the six cadavers.
14.2 Total body potassium (TBK)
A constant fraction (0.012%) of potassium exists in the body as the radioactive isotope 40K (half‑life = 1.3 × 109y). This isotope emits a high-energy γ‑ray of 1.46MeV, allowing the amount of potassium in the body to be estimated by counting with a whole-body γ‑spectrometer with sodium iodide detectors (Forbes et al., 1961). The isotope occurs in low concentrations so that the background counts from external radiation (cosmic rays and local sources of ionizing radiation) must be minimized. Hence, the whole-body counter must be shielded from the background radiation with lead, steel, or concrete shielding. Counting times of at least 15min are normally required for adults, with proportionately longer times for children and infants. Such times may be a problem for ill patients. Note that the required equipment is expensive, requires sophisticated technical support: availability is limited. Calibration of the whole-body counter must be done carefully because the 40K count detected by the whole-body counter is a function of the total body potassium concentration, the geometric configuration of the subject, and internal absorption of the 1.46MeV by the subject. As a result, the counter must be calibrated to allow for differences in the body build of the subjects. Hansen and Allen (1996) achieved this by using a phantom containing a known amount of potassium (as KCl solution) and gender-specific correction factors for weight and height. These authors reported CVs of 1.5% for precision and 4.5% for accuracy for measurements on adults. However, the accuracy of their method has not been confirmed by the analysis of human cadavers.14.2.1 Application of 40K measurements
Potassium occurs almost exclusively as an intracellular cation, primarily in the muscle and viscera. Negligible amounts occur in extracellular fluid, bone, and other noncellular sites. Measurement of total body potassium can therefore be used as a marker for the body cell mass, and as an index of the fat-free mass in healthy subjects, on the assumption that the fat-free mass has a constant proportion of potassium. Body cell mass represents the total mass of cells in the body that consume oxygen and produce work (i.e., the metabolically active, energy-exchanging mass of the body); it is the nonfat cellular portion of tissues, of which the primary components are skeletal muscle, organ tissue mass, blood, and the brain (Wang et al., 2004). The estimates of fat-free mass generated from total body potassium measurements are accurate and precise at all life stages and in conditions with uncertain hydration status (Naqvi et al., 2018). Originally 40K measurements were converted from the total body potassium content into the fat-free mass using a value of 69.4mmol K per kg fat-free mass or 2.71g/kg fat-free mass. These values were derived from cadaver analysis. However, a single value for all subjects is now known to be inappropriate and no longer accepted. The potassium concentration of fat-free tissue is a function of age and sex, with both men and women losing on average 5% of their original total body potassium per decade (Figure 14.1). Women have a lower potassium concentration in the fat-free mass than men. Note the reduction in total body potassium with age and the large variation within each age group. The latter emphasizes that total body potassium alone is a poor predictor of fat-free mass unless age‑ and sex-dependent equations are used (Ribeiro & Kehayias, 2014).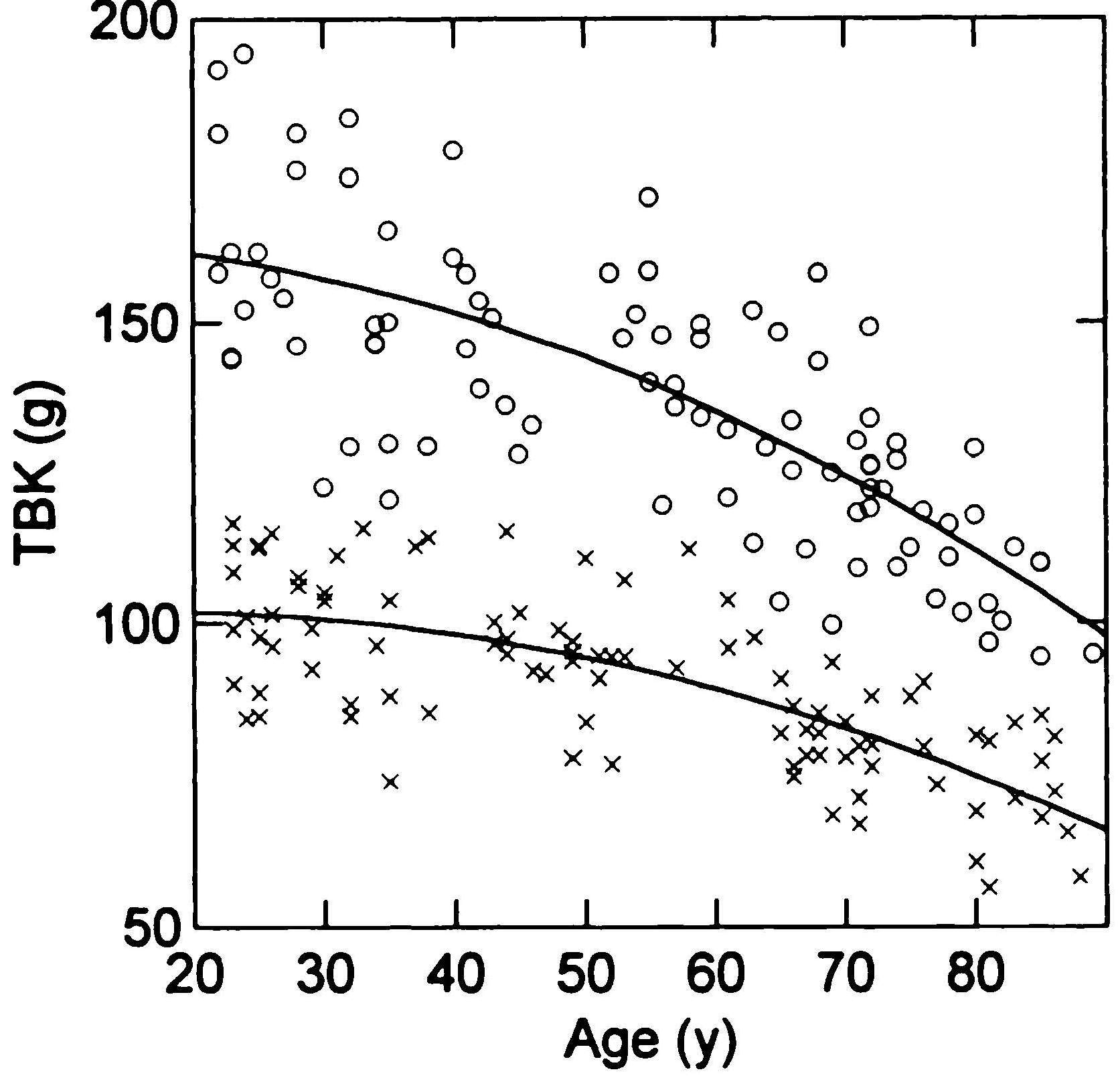
14.3 Total body water from isotope dilution
Either the stable isotope deuterium (2H), the radio-active isotope tritium (3H), or the stable isotope of oxygen (18O) can be used to measure total body water. Of the three, deuterium is now most frequently used. Standardized conditions are necessary for the measurement because fluid and food intake and exercise can all affect total body water concentrations. As a result, samples should be taken in the morning, after an overnight fast and the bladder has been emptied, and with a restriction of fluid intake. A tracer dose of sterile water labeled with an accurately known amount of the isotope (often deuterium oxide, D2O) is administered either orally or intravenously to the subject and allowed to equilibrate. Two samples of serum, or urine, or saliva are collected; one prior to the administration of the tracer and a second after the isotope has equilibrated with the subject's water pool (usually 3‑5h post-dose). The baseline sample measures the naturally present isotope in the total body water. The enrichment observed in the post-dose sample allows calculation of the total body water. The measurement of enrichment appears to be less reliable in urine than in serum probably because of the longer equilibration period of the bladder contents relative to blood. Ideally, no food or water is permitted during equilibration, which may take 3‑5h, depending on the isotope, the physiological sample, and the health condition of the patient. If fluid has been taken during the equilibrium period, a correction can be made, where necessary, to derive actual body water (Gutiérrez-Marín et al., 2019). Longer equilibration periods are necessary for urine compared to blood serum samples, for obese patients (Schoeller et al., 1980), or for those with edema, ascites, and shock (McMurrey et al., 1958). Table 14.2 demonstrates that the method is relatively robust and, independent of the different physiological fluids used.Samples (A) & (B) | Isotope | n | *Ratio | SD |
---|---|---|---|---|
(A) Saliva at 4h (B) Serum at 4h | 18O | 33 | 1.006 | 0.019 |
(A) Urine at 6h (B) Serum at 6h | 18O | 11 | 1.012 | 0.027 |
(A) Urine at 12h (B) Serum at12 h | 18O | 14 | 1.006 | 0.010 |
(A) Saliva at 3h (B) Saliva at 4h | 18O | 20 | 0.997 | 0.005 |
(A) Saliva at 3h (B) Saliva at 4h | 2H | 43 | 0.996 | 0.007 |
14.3.1 Application of body water measurements by isotope dilution
The measurement of total body water in both healthy and diseased persons is the most important application of isotope dilution techniques. All body water is present in the fat-free mass. Hence, total body water measurements can be used to estimate the fat-free mass. This estimation requires an assumed value for fat-free mass hydration, as shown below: \[ \small \mbox {Fat-free mass (kg) = (total body water (kg)) / hFFM }\] where hFFM = hydration of the fat-free mass. Based on the 2‑component model, once the fat-free mass has been determined, total body fat (TBF) and percentage of body fat can be calculated as shown below: \[ \small \mbox {Total body fat (kg) = body weight (kg) − fat-free mass (kg) }\] \[ \small \mbox {% body fat = (Total body fat (kg) × 100%) / body weight (kg) }\]Age | Males Hydration (%) | Females Hydration (%) |
---|---|---|
5y | 76.5 | 76.7 |
7y | 76.1 | 75.5 |
9y | 75.7 | 75.1 |
11y | 75.3 | 75.0 |
13y | 75.0 | 74.6 |
15y | 74.4 | 74.1 |
17y | 73.7 | 73.7 |
19y | 73.4 | 73.6 |
Author | Hydration constant | FFM (kg) | FM (kg) | FM (%) |
---|---|---|---|---|
Siri (1961) | 0.724 | 62.2 | 13.8 | 18.2 |
Van Raaj et al. (1988) | 0.740 | 60.8 | 15.2 | 20.0 |
Fidanza (1987). | 0.752 | 59.8 | 16.2 | 21.3 |
Catalano et al. (1995) | 0.762 | 59.1 | 16.9 | 22.3 |
14.4 Multiple dilution methods
Multiple dilution can be used to estimate the volume of various body fluid compartments that, in turn, can be used to estimate two components of the fat-free mass: extracellular mass (ECM) and the body-cell mass (BCM). Typically, multiple dilution involves determining both total body water (via isotope dilution; Section 14.3) and extracellular water (ECW), the latter using a tracer such as bromide that does not enter the intracellular space (Wong et al., 1989). The difference between these two measurements (i.e., TBW − ECW) reflects the intracellular water (ICW), which is more metabolically active than ECW and provides an estimate of BCM (Ribeiro & Kehayias, 2014). To estimate BCM using this approach, a pre-dose blood or urine sample is taken followed by dosing with deuterium oxide and sodium bromide solution. After a 3‑5h equilibration period, a final post-dose blood or urine sample is collected, although longer equilibration periods are needed for individuals with expanded ECW, including those with extreme obesity. Deuterium enrichment of the biological sample is described in Section 14.3, whereas bromide enrichment is determined by high-performance liquid chromatography or non-destructive liquid X‑ray fluorescence. The reported precision for ICW calculated using this approach is about 2.5% provided standardized protocols are used (Earthman, 2015). The ratio of ECW / TBW can also be calculated using this approach. In a study of nursing home elderly, the ratio ECW / TBW was significantly higher compared to the ratio in free-living elderly, prompting the suggestion that this ratio may have potential as a surrogate method for the clinical assessment of frailty (Kehayias et al., 2012).14.4.1 Application of multiple dilution methods
The ECM is defined as the component of the fat-free mass which exists outside the cells. It consists of both fluid (e.g., extracellular fluids, plasma volume) and solid (e.g., skeleton, cartilage, tendons) components which are involved in transport and support and are not metabolically active. In contrast, the BCM is the total mass of cells in the body that consume oxygen and produce work (i.e., the metabolically active, energy-exchanging mass of the body). These components are the nonfat cellular portion of tissues, primary the skeletal muscle, organ tissue mass, blood, and the brain (Wang et al., 2004). Nutritional status, physical activity level, and disease states alter BCM, which thus serves as a helpful biomarker.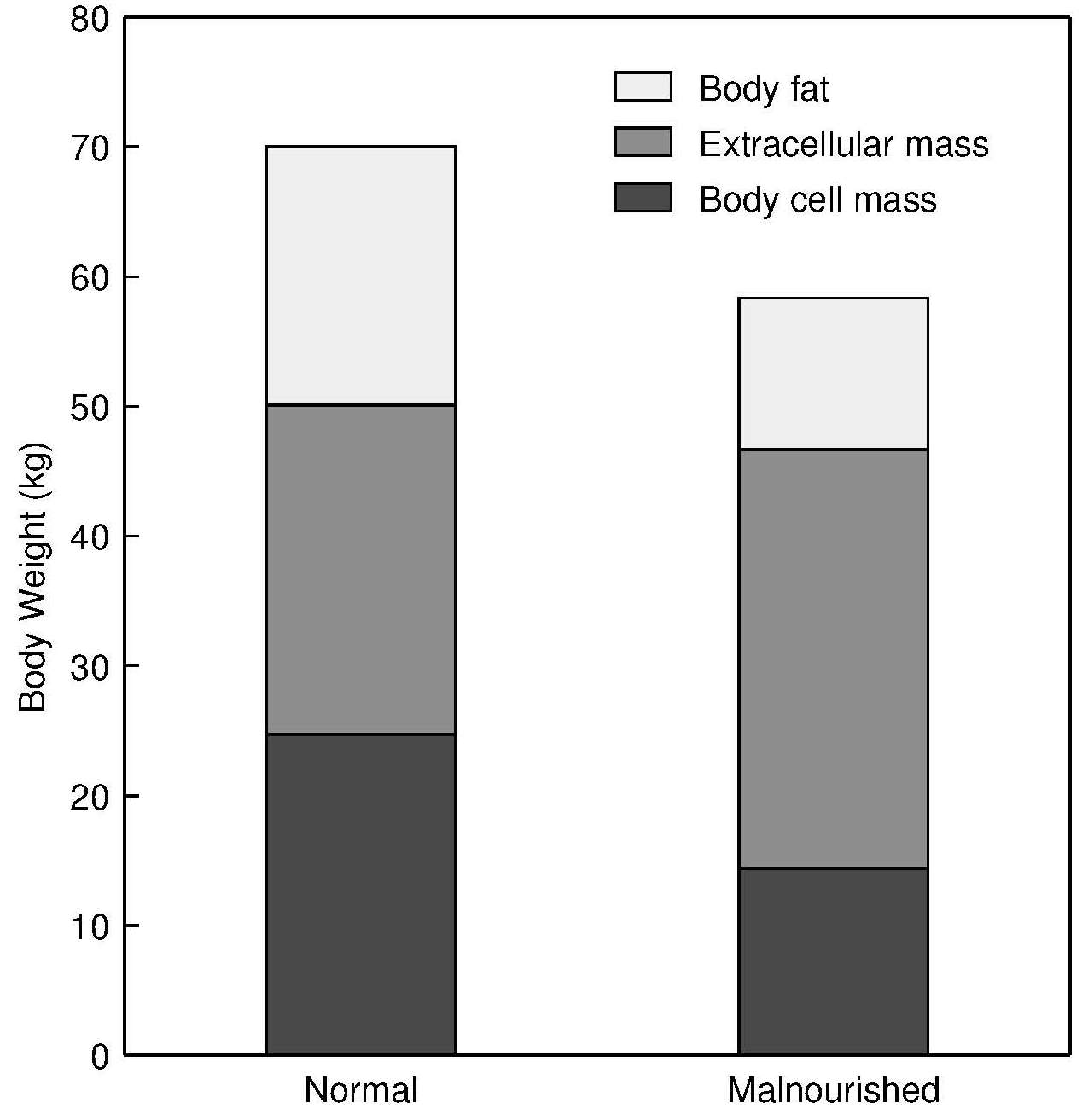
14.5 In vivo activation analysis
A group of related techniques involving in vivo neutron activation analysis (NAA) allow the direct estimation of the amount of a range of chemical elements in the living human body. Most other techniques used in body composition studies, with the exception of whole body counting for potassium, generate data on tissue density or volume, but not data on the amount of a component. As a result, multicomponent elemental models based on in vivo NAA have gradually become accepted as reference methods for the calibration of many of the other techniques described in this chapter. Nearly all the major elements present in the body can be analyzed by in vivo NAA, including hydrogen, oxygen, carbon, nitrogen, calcium, phosphorus, sodium, and chlorine (Cohn et al., 1984). Of special interest is the application of NAA to measure the carbon-to-oxygen (C/O) ratio in vivo . This ratio can provide an independent, unbiased measure of the distribution of fat and fat-free mass, which is not dependent on the assumptions about the composition of fat-free mass. Small changes in fat-free mass can be monitored, making the method appropriate for studying the depletion of fat-free mass with aging (Kehayias et al., 2000). A major negative factor associated with in vivo NAA is that the subject is exposed to radiation. This and the associated risks must always be explained to the subject. In vivo NAA is most used in clinical medicine for the determination of total body nitrogen (TBN), total body calcium (TBCa), and bone mass as discussed below. TBN and TBCa are only determined in a relatively small number of laboratories worldwide — an indication of the expense involved and technical difficulties associated with the method.14.5.1 Total body nitrogen by in vivo NAA
Nitrogen is normally determined by NAA by bombarding the patient, in a supine position, with a low neutron flux from a 238PuBe source or from a cyclotron or neutron generator. During irradiation, a proportion of 14N is converted to an excited state of 15N, which decays almost immediately to its ground state, emitting a “prompt” γ‑ray at 10.83MeV. This activity is counted by an array of sodium iodide detectors in a whole body counter (Figure 14.5).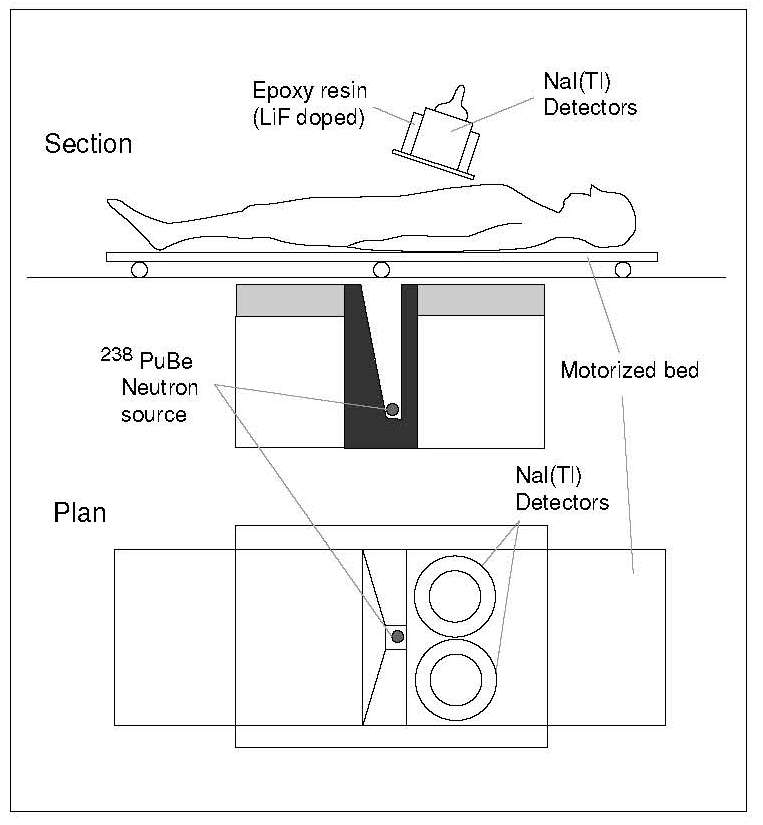
14.5.2 Application of body nitrogen by in vivo NAA
Changes in total body protein of hospital patients with diseases such as cancer, renal dysfunction, hypertension, chronic heart disease, and rheumatoid arthritis, and with severe trauma or sepsis have been studied using prompt γ‑neutron activation (Beddoe & Hill, 1985). The results indicate that substantial losses of body protein may occur, even when conventionally adequate nutritional support has been provided for some of these patients. Depleted total body protein is also a consistent finding among acutely ill anorexic patients. In a longitudinal study, Haas et al. (2018) reported that after recovering from anorexia nervosa, depletion of body protein measured by in vivo INAA remained in adolescent patients after 7mos, even though body weight was restored. Clearly, further work is required to identify nutritional intervention procedures which minimize loss of body protein in hospital patients and those with anorexia nervosa.14.5.3 Total body calcium by in vivo NAA
The method is based on the conversion of a proportion of the naturally occurring isotope 48Ca in the body to 49Ca (half-life = 8.8min) by exposing the patient to a low neutron flux. Immediately following irradiation, the patient is transferred to a whole-body counter, and the γ‑rays emitted by the decay of 49Ca are detected by an array of sodium iodide detectors. The counting geometry is similar to that used for total body potassium, and again care must be taken in positioning the subject and correctly accounting for the varying height, weight, sex, and body mass index of subjects. In particular, for subjects with BMI > 30, the effects of neutron attenuation become significant, necessitating additional corrections or a special calibration (Ma et al., 2000). The reported accuracy and precision are from 1‑2% (Cohn et al., 1974).14.5.4 Application of total body calcium by in vivo NAA
The radiation exposure using this method varies from 2.5‑25mSv, depending on the neutron source. This range is considerably higher than that experienced during dual X‑ray absorptiometry (DXA) and limits the general applicability of the method. Consequently, the use of neutron activation analyses for the assessment of total body calcium has largely been replaced by DXA (Section 14.11). Instead, the method is now mainly used as a calibration tool for other techniques. The assessment of total body calcium by in vivo neutron activation analysis is also discussed in Chapter 23 under the assessment of calcium status.14.6 Densitometry
Body density was one of the first measures of body composition to be made Behnke et al., 1942). It is relatively easy to measure, and in the past, body density was the gold-standard method to determine percentage body fat using the 2‑component model. Certain disease states characterized by excess fluid retention and under-mineralization decrease the density of fat-free mass. Consequently, densitometry is now often combined with other measures in a 4‑component model of body composition so that hydration and density of fat-free mass are measured along with the actual bone mineral content, thus providing a more accurate assessment. (Silva er al., 2013). Hydrostatic weighing was the initial densitometric method used to determine body volume, and hence whole body density. However, it is now being replaced by plethysmographic methods that are more acceptable to subjects, particularly children (Fields & Goran, 2000; Wells & Fewtrell, 2006). All three methods are described below. A concluding section describes the calculation of body fat from body density (Section 14.6.4).14.6.1 Hydrostatic weighing
The conventional method of directly measuring whole body density involves weighing the subject and then using Archimedes' principle to determine the volume of the subject. Thus, the subject is weighed first in air and then when completely submerged in water in a large tank. The subject is instructed to squeeze out any air bubbles trapped inside the bathing suit, and to expel as much air as possible from the lungs before immersion. The hydrostatic weight is recorded at the end of the forced expiration. Multiple readings should be taken using a continuous and sensitive recording of underwater mass, the heaviest corresponding to the most complete expiration. This method requires a high degree of water confidence and thus the method is not suitable for children younger than 8y, the elderly, obese, or unhealthy persons. The body volume is then calculated from the apparent loss of weight in water (i.e., the difference between the weight of the person in air and his or her corresponding weight in water). Once total body mass and body volume have been determined, whole body density can be readily calculated, on the basis that density is mass per unit volume and the density of water is l.0kg/L at 4°C: \[ \small \mbox { Whole body density = (body weight in air (kg)) / (apparent loss in weight (kg)) }\] Three corrections must be applied:- Hydrostatic weighing is usually performed in water at 30°C instead of 4°C. At this higher temperature, the density of water is 0.9957kg/L, and a water temperature correction factor must be applied.
- Air trapped in the lungs also contributes to the amount of water displaced by the subject under water. Residual air in the lungs can be measured while the subject is in the tank. Alternatively, the volume can be estimated using a nitrogen washout, helium dilution, or oxygen dilution (Brodie, 1988). This correction can also be estimated from empirical equations. The residual air volume is then subtracted from the body volume.
- The volume of the air trapped in the gastrointestinal tract also contributes to the total amount of water displaced. This volume is small and is never measured. It is often taken as 100mL.
14.6.2 Water-displacement plethysmography
The use of a plethysmograph eliminates the necessity for totally immersing the subject in water, a disadvantage of hydrostatic weighing (Section 14.6.1). For the measurement, the plethysmograph is zeroed and filled with water (Figure 14.6).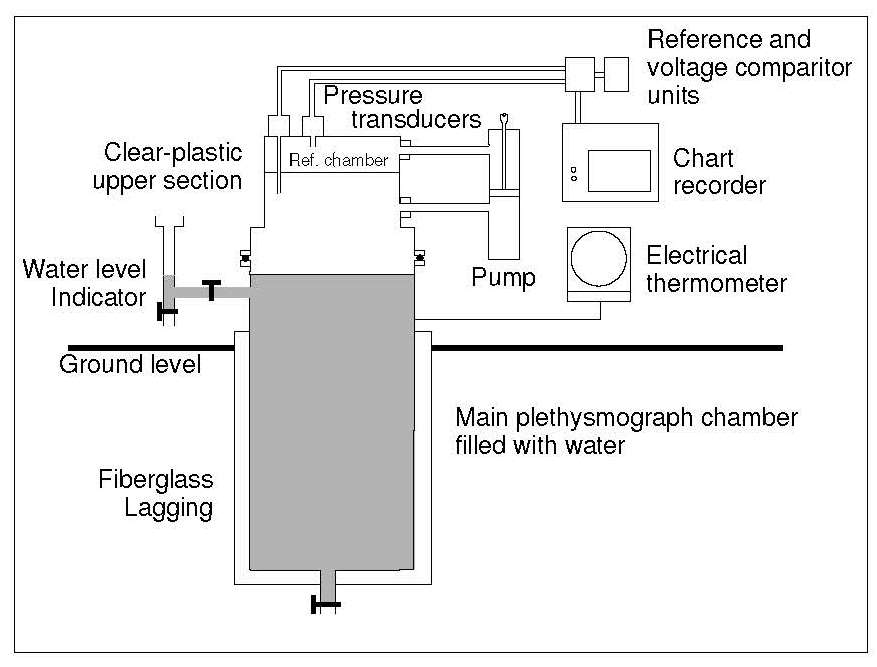
14.6.3 Air-displacement plethysmography
The measurement of body volume has been significantly eased by the development of an air-displacement plethysmography device (Bod Pod). These devices determine the volume of a subject indirectly by measuring the volume of air displaced by the subject inside an enclosed chamber — plethysmograph or Bod Pod. The method is quick, comfortable, automated, non-invasive, safe, and does not require extensive technical training. For infants up to about 6mos of age, a device known as the “Pea Pod” is available and can accommodate infants up to about 8kg. For small children from 2‑6y, the Bod Pod with a Pediatric Option can be used, whereas the standard Bod Pod can be used for older children (i.e., > 6y), adults, and the elderly. The air-displacement plethysmograph consists of an ovoid fiberglass structure divided into two sections: a rear reference chamber and a front test chamber containing the seated subject (Figure 14.7).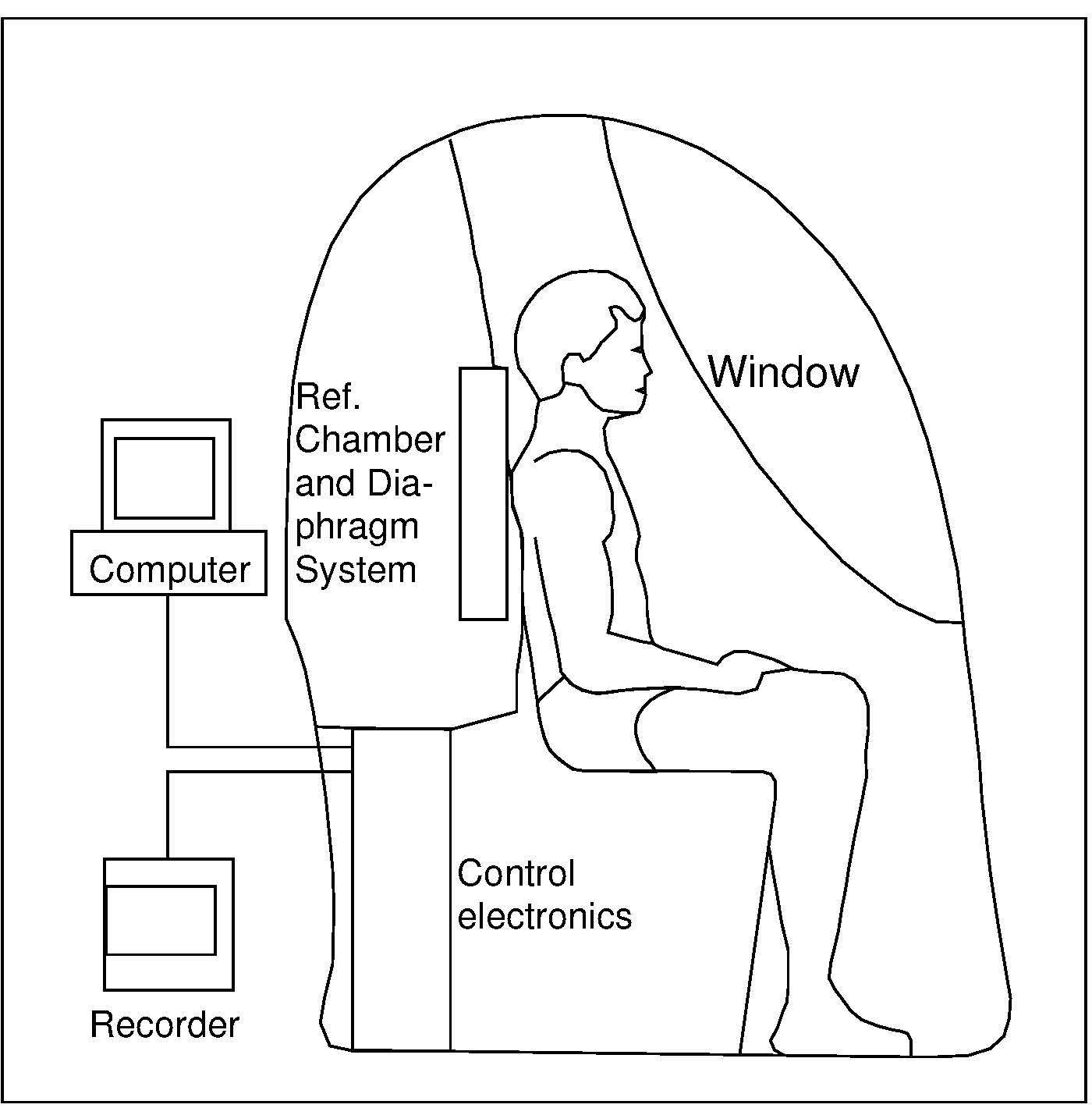
14.6.4 Application of densitometry to calculate body fat
Once whole-body density has been measured by one of the methods outlined above, the percentage body fat can be calculated. This involves the selection of an empirical densitometric equation relating fat content to whole body density (D). Several empirical densitometric equations have been derived based on the classic two-component model for body composition. As noted earlier, in this model body weight is divided into fat and fat-free mass, and relies on assumptions that ignore inter-individual variability in the composition of the fat-free mass. All the classical densitometric equations shown below assume:- The density of the fat-free mass is relatively constant.
- The density of fat for normal persons does not vary among individuals.
- The water content of the fat-free mass is constant.
- The proportion of bone mineral (i.e., skeleton) to muscle in the fat-free body is constant.
Males | Females | |||||||
---|---|---|---|---|---|---|---|---|
Age | Hydra- tion (%) |
Density (kg/L) | C1 | C2 | Hydra- tion (%) | Density (kg/L) | C1 | C2 |
5y | 76.5 | 1.0827 | 5.36 | 4.95 | 76.7 | 1.0837 | 5.33 | 4.92 |
6y | 76.3 | 1.0844 | 5.32 | 4.90 | 76.1 | 1.0865 | 5.27 | 4.85 |
7y | 76.1 | 1.0861 | 5.28 | 4.86 | 75.5 | 1.0887 | 5.22 | 4.79 |
8y | 75.9 | 1.0877 | 5.24 | 4.82 | 75.2 | 1.0900 | 5.19 | 4.76 |
9y | 75.7 | 1.0889 | 5.21 | 4.79 | 75.1 | 1.0909 | 5.17 | 4.74 |
10y | 75.5 | 1.0900 | 5.19 | 4.76 | 75.0 | 1.0916 | 5.15 | 4.72 |
11y | 75.3 | 1.0911 | 5.16 | 4.73 | 75.0 | 1.0924 | 5.13 | 4.70 |
12y | 75.2 | 1.0917 | 5.15 | 4.72 | 74.9 | 1.0937 | 5.10 | 4.67 |
13y | 75.0 | 1.0920 | 5.14 | 4.71 | 74.6 | 1.0954 | 5.07 | 4.63 |
14y | 74.8 | 1.0927 | 5.13 | 4.69 | 74.4 | 1.0975 | 5.02 | 4.58 |
15y | 74.4 | 1.0942 | 5.09 | 4.66 | 74.1 | 1.0996 | 4.98 | 4.53 |
16y | 74.0 | 1.0960 | 5.05 | 4.61 | 73.8 | 1.1011 | 4.95 | 4.49 |
17y | 73.7 | 1.0978 | 5.02 | 4.57 | 73.7 | 1.1020 | 4.93 | 4.47 |
18y | 73.5 | 1.0991 | 4.99 | 4.54 | 73.6 | 1.1027 | 4.92 | 4.46 |
19y | 73.4 | 1.1000 | 4.97 | 4.52 | 73.6 | 1.1031 | 4.91 | 4.45 |
20y | 73.3 | 1.1006 | 4.96 | 4.51 | 73.6 | 1.1035 | 4.90 | 4.44 |
14.7 Total body electrical conductivity
Total body electrical conductivity (TOBEC) is measured by observing the changes induced by placing the subject in an electromagnetic field Baumgartner, 1996). The extent of the change depends on the overall electrical conductivity of the body and, in particular, on the proportions of fat and the fat-free mass in the body: the fat-free mass, comprised largely of water with dissolved electrolytes, will readily conduct an applied electric current, whereas fat is anhydrous and a poor conductor. In more modern equipment, the subject lies supine on a motorized bed (Figure 14.9) that is passed in a series of steps progressively through a uniform solenoid coil.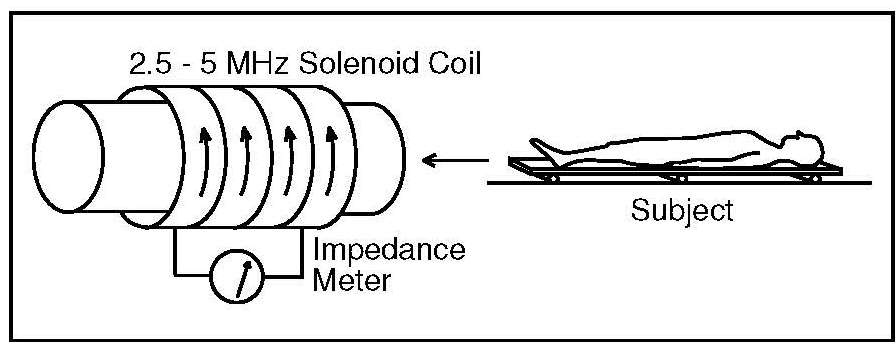
14.7.1 Applications of total body electrical conductivity
To interpret TOBEC measurements in terms of the quantity of fat-free mass in the body, a calibration equation is applied generated by measuring the fat-free mass of a reference population using an alternative technique and relating this to the TOBEC value of each individual. A calibration equation has also been developed to measure fat-free mass and fat reliably and precisely in neonates through 1y of age (Fiorotto & Klish, 1991; Fiorotto et al., 1995). TOBEC is relatively insensitive to shifts of fluid or electrolytes between the intracellular and the extracellular compartments and to variations in bone mineralization. For example, in a study of middle aged and elderly subjects (35‑90y), higher values for fat-free mass were reported when predicted from measurements based on TOBEC compared to those for fat-free mass predicted by using densitometry or hydrometry (Van Loan & Koehler, 1990). These findings led to the suggestion that the higher fat-free mass values arise because the TOBEC signal is unaffected by decreases in bone mineralization.14.8 Bioelectrical impedance
The use of bioelectrical impedance (BIA) for the assessment of body composition is widespread, in large part due to the affordability, portability, and ease of use of the bioimpedance devices. None of the BIA devices measure body composition directly. They measure an electrical response of the body, resistance, when exposed to an electrical current. The resistance measured is transformed into a prediction of total body water by an algorithm, and from that the body fat mass is determined. Three categories of BIA devices are available commercially: single-frequency (SF‑BIA), multiple-frequency (MF‑BIA), and bioimpedance spectroscopy (BIS); these are described below together with some new developments in BIA. Both the SF‑BIA and MF‑BIA devices rely upon population-specific prediction equations. In contrast, BIS uses biophysical modeling to estimate body compartments. All the algorithms are based on or validated against other body composition reference methods, which are not totally accurate and error-free. Each BIA device works with an inbuilt algorithm specific to the device and for the population for which the algorithm was developed so it is not possible to compare studies unless the same combination of device / equation / population is used (Ward, 2019; Sheean et al., 2020). Furthermore, the reference population on which the BIA algorithm was based must be appropriate for the target subject being measured (Lemos & Gallagher, 2017). Many factors may influence the precision and accuracy of BIA techniques. They include factors associated both with the individual (e.g., degree of adiposity, fluid and electrolyte status, skin temperature) and with the environment (ambient temperature, proximity to metal surfaces and electronic devices), the assumptions underlying prediction or modeling equations, instrumentation, and variations in the protocols used for the BIA measurements. Box 14.2 presents recommendations for optimizing whole body BIA measurements.- Food/beverage and activity. Individual should fast (no food that day except water) and avoid alcohol, caffeine, and exercise for at least 8h prior to measurement in the morning (research settings); shorter time frames and other times of day may be acceptable in the clinical setting — note time of day for consistency in follow-up measures.
- Void bladder. Individual should void bladder prior to measurement.
- Clean skin surface. Clean skin surface well with alcohol; individual should not use lotion or oils on the skin prior to measurement; avoid placing electrodes on broken skin.
- Device calibration. Calibrate the bioimpedance device according to the manufacturer’s recommendations prior to measurement.
- Height and weight. Obtain an accurate measure of height and weight.
- Device placement. Place device on a nonmetal surface, at least 1m away from electronic or magnetic devices.
- Ambient temperature. Avoid excessively warm or cool ambient temperature.
- Electrodes and leads. Use electrodes with sufficient surface area (≥ 4cm2); store electrodes in sealed bag away from heat; use device-specific leads provided by manufacturer.
- Electrode placement. Place electrodes at least 5cm apart, if possible; proximal electrodes should never be moved from standard anatomical site placement; if necessary, the distal electrodes may be moved to achieve at least 3cm of separation; the most important thing is to measure and record distance between electrodes to ensure placement consistency for follow-up measurements.
- Side of body. If using standard tetrapolar placement of electrodes, measure on the same side of the body as previous measures; in individuals with amputations, muscle atrophy, or other abnormal conditions, use the nonaffected side, if possible; be consistent on side of measurement for follow-up. Right-side measurements are commonly used in the literature.
- Body positioning and limb separation. Body position should be supine, except for stand-on scale devices, with arms separated ≥30° from the trunk and legs separated by about 45°; in individuals with overweight and obesity, separate arms from trunk and legs from each other using rolled cotton towels/blankets.
- Fluid and electrolyte status. Note if serum electrolytes are abnormal; it is best to conduct bioimpedance measurements only when serum electrolytes are normal. Note if edema is present; edema causes lower resistance values.
- Menstrual cycle in women. Note menstrual cycle; be consistent in terms of timing for follow-up measurements.
- Timing of measurement. If individual is ambulatory, individual should assume a supine position for 5‑10min; standardize the timing for measurements by noting the time when the individual assumes the supine position and the time when you take the measurement (eg, at 10 minutes), and ensure consistency of timing for all followup measurements. Note if individual is confined to bed.
- Repeat measurements. Repeat measures recommended for research studies.
14.8.1 Single-frequency bioelectrical impedance (SF‑BIA)
Single frequency BIA devices (SF‑BIA) were the earliest devices developed. With these, the impedance of a weak electrical current (typically 500‑800mA) and a single current frequency (i.e., typically 50kHz) is passed between two electrodes, usually located in the standard wrist-ankle tetrapolar arrangement on one side of the body, as shown in Figure 14.10, while the subject is supine in a horizontal position.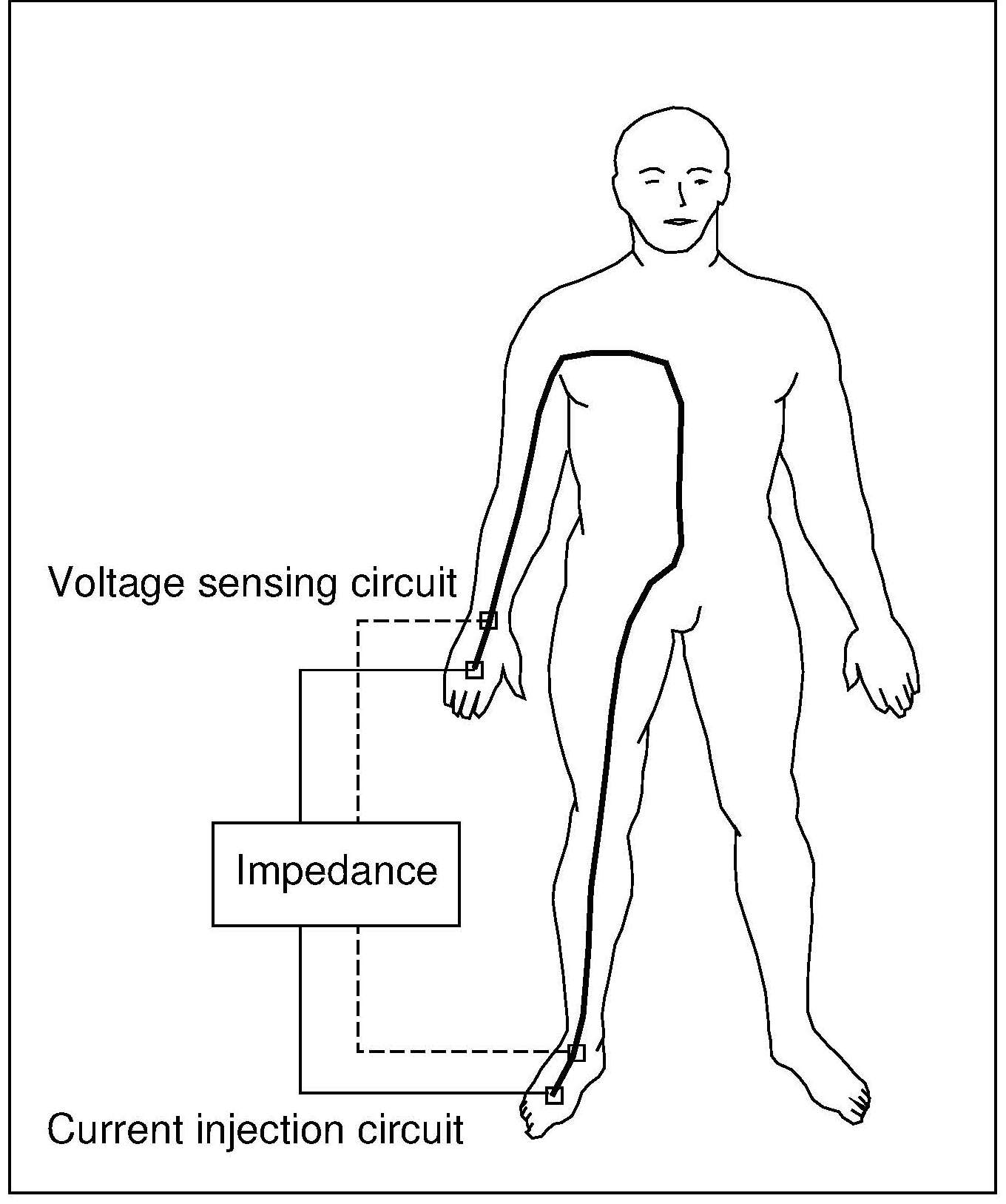
14.8.2 Multiple-frequency bioelectrical impedance (MF‑BIA)
Multiple-frequency BIA devices (MF‑BIA) measure impedance at two or more frequencies, typically at 4 or 5 frequencies, including at least one low (most commonly 5kHz) and several higher ones (typically 50, 100, 200, and 500kHz). At lower frequencies the impedance to current flow allows for the determination of the extracellular water space (ECW), whereas at the higher frequencies (i.e., > 50kHz) the impedance to current allows for the determination of ECW and intracellular water space (ICW) (i.e., TBW). The volume of ICW, derived by subtracting ECW from TBW, can be used to estimate body cell mass (BCM) based on the assumption that cells are comprised of 70% water. Several population-specific prediction equations are available to predict ECW, TBW, ICW, and BCM based on reference values derived from appropriate criterion methods. Nevertheless, again underlying assumptions may be violated in those individuals with obesity and in certain clinical populations, leading to errors, highlighting the importance of not accepting, without question, the output from a BIA device which uses the proprietary equations of the manufacturer (Earthman, 2015). Sex-specific BIA prediction equations, validated earlier from MF‑BIA resistance measures in the U.S NHANES III in 1988‑1994 (Chumlea et al., 2002), have been used to compile ratios of fat mass to fat-free mass at 5th, 50th, and 95th percentiles by sex, age group, and BMI category (i.e., underweight, normal weight, overweight, class I/II and class III obesity) for non-Hispanic persons aged 18‑90y. These fat/fat-free mass reference values that account for age, sex, and BMI can be used to identify individuals at risk for body composition abnormalities (Xiao et al., 2018). Measurements from newer MF‑BIA devices can now be made across body segments such as the limb and trunk, or across a small body region such as the muscle bed of the calf. Some MF‑BIA devices can be used in upright and supine positions so that they can be used for non-ambulatory or bed-bound persons.14.8.3 Bioelectrical impedance spectroscopy (BIS)
Bioelectrical impedance spectroscopy (BIS) devices use biophysical modeling to estimate body compartments, as noted earlier; see Mulasi et al. (2015) for more details. These devices apply the electric current (typically ≤ 800µA) over a range of frequencies, from very low (e.g., 1 or 5kHz) to very high (e.g., 1000‑1200kHz), measuring impedance data at ≥ 50 frequencies. This allows a more direct and individualized measure of ECW, ICW, and TBW compartments compared with those generated with SF‑BIA and MF‑BIA. Such measures are particularly useful in patients with altered fluid homeostasis (Mulasi et al., 2015). For those with obesity, the values for ECW and ICW can now be corrected using BMI as a surrogate for adiposity, thus allowing a more accurate assessment of body composition in individuals with obesity (Moissl et al., 2006). Many validation studies of BIS conducted in healthy and clinical populations are available. Although good agreement at the population level can be achieved, agreement between reference methods and BIS at the individual level is poor, as noted for the measurements from SF‑BIA and MF‑BIA devices. This limits the assessment of whole body masses and fluid volumes in clinical settings.14.8.4 Bioelectrical impedance vector analysis (BIVA)
Bioelectrical impedance vector analysis is a graphical procedure which uses the plot of resistance and reactance standardized for height to create a vector that can be compared with gender‑ and race-specific reference values from healthy population samples. BIVA can be generated from 50kHz data. It can provide information on hydration and body cell mass for patients in whom calculation of body composition is incorrect due to altered hydration (Mulasi et al., 2015). In a recent study of children with severe-acute undernutrition in Ethiopia, BIVA measurements successfully differentiated between those children who were dehydrated and those with edema (Girma et al., 2021). During treatment, edematous children lost fluid whereas non-edematous children gained small amounts of fat-free tissue. Moreover, BIVA parameters correlated with biomarkers of nutritional status (Girma et al., 2018).14.8.5 New developments in bioelectrical impedance analysis
The questionable validity of BIA approaches for the assessment of whole body composition estimates in clinical populations, especially those with abnormal body geometry or altered fluid homeostasis, has led to the use of raw BIA measurements per se for bedside assessment of nutritional status and/or clinical outcomes. Raw BIA data are independent of the use of regression prediction models and assumptions of constant chemical composition of the fat-free mass, unlike the measurements from SF‑BIA and MF‑BIA devices. The raw BIA data used comprise single-frequency (50kHz) phase-sensitive measurements to determine: (i) phase angle, and (ii) the ratio of multifrequency impedance values. Phase angle is estimated directly by a phase-sensitive BIA device without additional conversion to specific body compartments, followed by comparison with population-specific reference values. Phase angle is also independent of the body weight and height of an individual patient. The phase angle concept is based on changes in resistance and reactance as alternating currents pass through evaluated tissues, providing information on hydration status and cell mass; for more details see Lukaski et al. (2017). Low phase angles values are typically related to more-severe illnesses and worse overall health outcomes. Currently, the major challenge of using phase angle for clinical assessment is the lack of consensus on the choice of cut-points to identify malnutrition (or poor clinical outcomes) (Mulasi et al., 2015). The ratio of impedance measured at 200kHz to impedance measured at 5kHz — termed the impedance ratio or prediction marker — is said to reflect the ratio of ECW/TBW fluid distribution, and which is currently being explored as a potential indicator of nutritional status and/or clinical outcomes. However, as stated for phase angle, the lack of a consensus on the reference cut-points to use to identify malnutrition (or poor clinical outcomes) remains a major challenge for the use of the impedance ratio for clinical assessment (Mulasi et al., 2015). investigations to date suggest that such reference cut-points may differ depending on the population under study and the BIA device used. Of particular interest is whether both phase angle (PA) and the impedance ratio (IR) are useful for the diagnosis of sarcopenia (with and without the presence of obesity) and malnutrition in clinical settings (Mulasi et al., 2015). Reference cut-points for both phase angle and impedance ratios by sex, ethnicity, and age-decade have been compiled from U.S NHANES 1999‑2004 based on BIS data. Validation with other reference measures (e.g. DXA) is needed to assess whether PA/IR are appropriate for the assessment of nutritional status in a clinical population (Kuchnia et al., 2017).14.8.6 Applications of bioelectrical impedance
Several population-specific regression equations have been developed based on reference methods capable of predicting not only fat-free mass, but fat mass and other compartments from 50kHz data. Examples of these reference methods include those based on a 4‑component model or dual-energy X‑ray absorptiometry (DXA); see Kyle et al. (2004) for more details. The regression equation chosen must be matched closely to the characteristics of the subject. However, unfortunately, many devices do not specify the equation programmed into their software so that the prediction equation used with the device may not be appropriate for the individual being measured. Use of a fat-free mass index has also been explored for its potential to predict nutritional status and/or clinical outcomes. The index is generated from 50kHz data and is a height-corrected index of fat-free mass (i.e., fat-free mass/height squared) calculated by a standardized prediction equation and compared with reference data (Schutz et al., 2002). To date, challenges remain when applying fat-free mass index to assess nutritional status in clinical settings and more research is needed (Mulasi et al., 2015). Interestingly, the American Society for Parenteral and Enteral Nutrition (ASPEN), does not support the use of BIA for the assessment of body composition in the clinical setting, based on their systematic review of 23 BIA studies. Their main objections included the scarcity of data on the validity of BIA in specific clinical populations, difficulties comparing studies using different BIA devices, variability in the body compartments estimated, and the proprietary nature of manufacture- specific BIA regression models to procure body composition data. For a summary and discussion of the studies reviewed which led to this conclusion, see Sheean et al. (2020).14.9 Computerized tomography
Computerized tomography (CT) is a high-resolution imaging technique that is widely used in clinical settings to quantify in vivo body composition at the tissue-organ level. Total adipose tissue, subcutaneous adipose tissue, and visceral adipose tissue can be assessed. Computerized tomography can also measure skeletal muscle, individual muscle, or muscle groups, and evaluate the quality of muscle by identifying the infiltration of fat within the muscle, a condition known as myosteatosis. Computerized tomography is especially useful for investigating quantitative and qualitative changes in muscle and fat in the trunk area where the use of DXA is limited. Computerized tomography (CT) is based on the relationship between the attenuation of an X‑ray beam and the physical density of the tissues through which the beam has passed. The known differences in attenuation of X‑rays between lean soft tissue and adipose tissue can be used to distinguish these tissues as well as to determine mixtures of them. From this relationship, a two-dimensional high-resolution radiographic image of the underlying anatomy of the scan area can be constructed. The CT scanner is made up of two components: a collimated X‑ray source and detectors, and a computer that processes the scan data and produces an X‑ray image. The subject lies on a movable platform within the scanner gantry. The designated area to be scanned is a plane through the middle of the central aperture of the gantry and parallel to the gantry. The X‑ray beam is made to rotate around the subject, generating a cross-sectional “slice” through the patient. As the X‑rays pass through the tissue, the beam undergoes attenuation, and the intensity is recorded and stored in the scanner computer. The latter then processes the stored information by using a series of complex algorithms to construct a cross-sectional image (Figure 14.11). Multiple images generated following the movement of the patient through the scanner gantry are used to produce an integrated scan of the subject.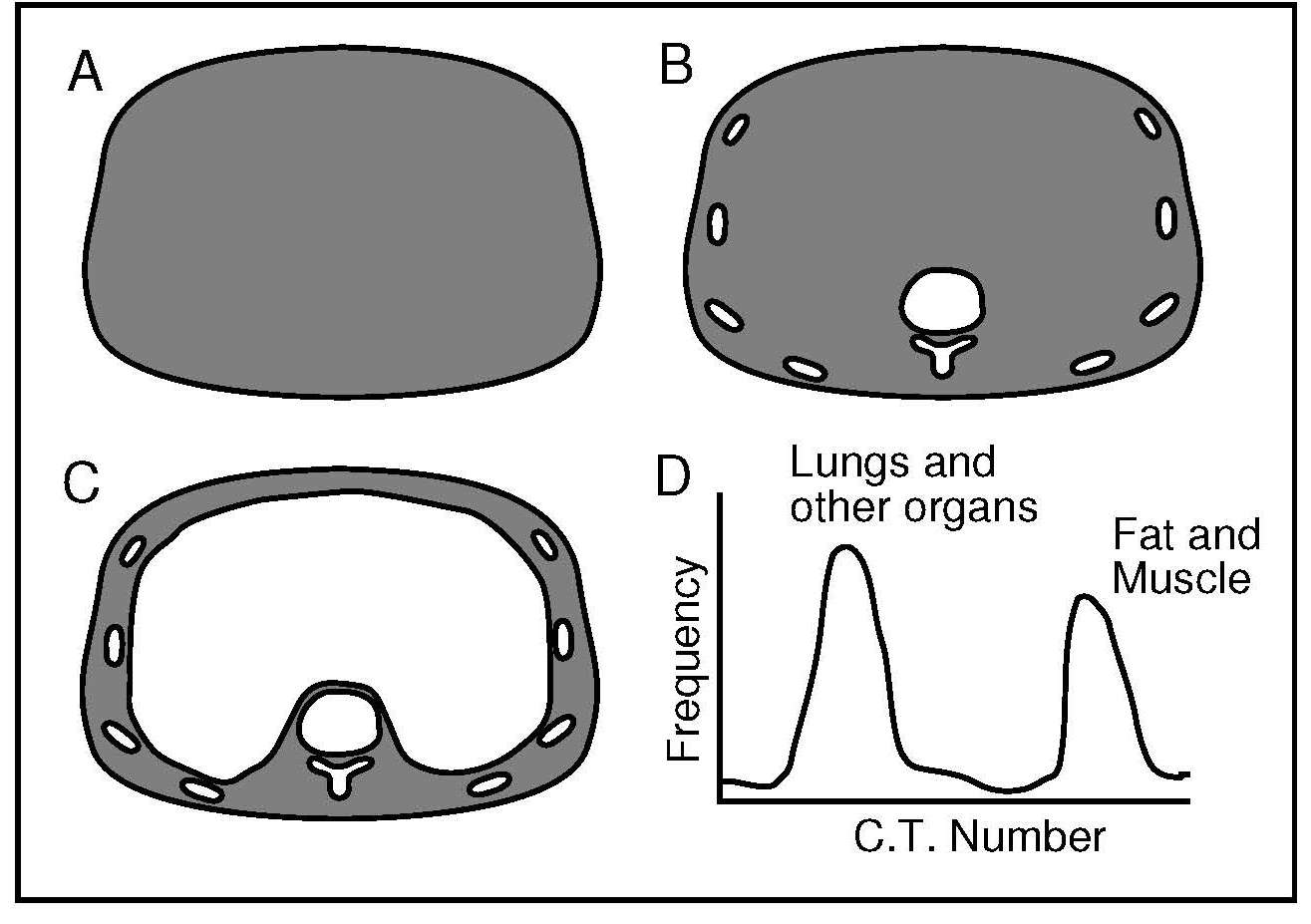
14.9.1 Application of computerized tomography
The method has several uses. It can be used to assess changes in the visceral organ mass in undernutrition and obesity, to portray the distribution of subcutaneous versus visceral adipose tissue, and to establish bone density in osteopenia (Heymsfield et al., 1987). New techniques with improved spatial resolution can be used to detect and measure fat in areas of the body where fat is not physiologically stored, such as the liver, pancreas, heart, and skeletal muscle. The fat deposited in these areas is termed visceral ectopic fat and is known to contribute to the development of coronary artery disease as well as other cardiovascular disorders (Neeland et al., 2019). The region of special interest for the study of body composition using CT scans is the third lumbar vertebra (L3). This is the region where a single image of a cross- sectional area provides the best correlation of whole-body skeletal muscle volume. The validity and accuracy of body composition measurements by CT based on cross-sectional area of tissues have been validated by studies of human cadavers, although no validation of the calculation of tissue volume has been performed (Fosbøl & Zerahn, 2015). Increasingly, CT scans are also being used to identify CT-defined sarcopenia, a condition associated with a decrease in muscle mass and function which, although originally described in the elderly, is also of concern among the chronically ill nonelderly (Peterson & Braunschweig, 2016). To date, however, there are no clear diagnostic cutoff values for CT to identify sarcopenia based on skeletal muscle mass, which has limited the application of CT for clinical use.14.10 Magnetic resonance imaging
Unlike computer tomography or DXA, magnetic resonance imaging (MRI) does not use ionizing radiation so that the technique can be used on infants, and for long-term follow-up when multiple scans on the same person are required. The MRI technique is mainly used to evaluate the quantity and distribution of adipose tissues and skeletal muscle mass, although it can also detect changes in body composition, even in the presence of small body weight changes (Lemos & Gallagher, 2017). In older equipment, scanning times of 10min were necessary, but in more modern equipment, this has been reduced to under 2min. Nevertheless, the MRI technique requires technical expertise, is expensive, and the equipment is bulky (Prada & Heymsfield, 2014). Magnetic resonance imaging uses differences in the nuclear magnetic resonance properties of hydrogen atoms in organic and non-organic environments to distinguish signals originating from fat, fat-free mass, and free water. The hydrogen protons behave slightly differently in adipose versus lean tissues. The differences are in the relaxation time that it takes for the nuclei to release the radio-frequency-induced energy and return to a random configuration. These differences can be used to map the distribution of adipose versus lean tissue in the body (Ross, 1996). The imaging process involves placing the subject in a very strong magnetic field. Some of the nuclei in the body attempt to align themselves relative to the applied field. The effect is particularly marked for 1H protons. Only a small fraction of the protons become aligned, but they are sufficiently numerous for the effect to be detectable when the field is removed or altered. It is then that the differences between the lean and adipose tissue become apparent.14.10.1 Application of magnetic resonance imaging
Magnetic resonance imaging is often used in clinical settings, when, instead of using whole body imaging which is time consuming and expensive, sectional body composition studies that often employ the L3 lumbar vertebra as the landmark, are used. This landmark has the highest correlation with whole body skeletal muscle and visceral fat volume. Alternatively, to identify sarcopenia in older adults, a single-slice at the mid-thigh level can be used as this provides a good estimate of skeletal muscle and fat volume in the thighs, and correlates with clinical criteria; see Lee et al. (2019) for more details.14.11 Dual energy X‑ray absorptiometry
Dual-energy X‑ray absorptiometry (DXA) is a technique that can be used with individuals (except pregnant women) across the entire age range and at relatively low cost. DXA is widely used to assess body composition, with an overall precision that exceeds that of any other body composition method (Prada & Heymsfield, 2014). With the latest generation of densitometers, body composition can be assessed with a single whole-body scan so that radiation exposure is low with a minimal acquisition time. In addition, the newer instruments enable individuals with extreme obesity to be scanned.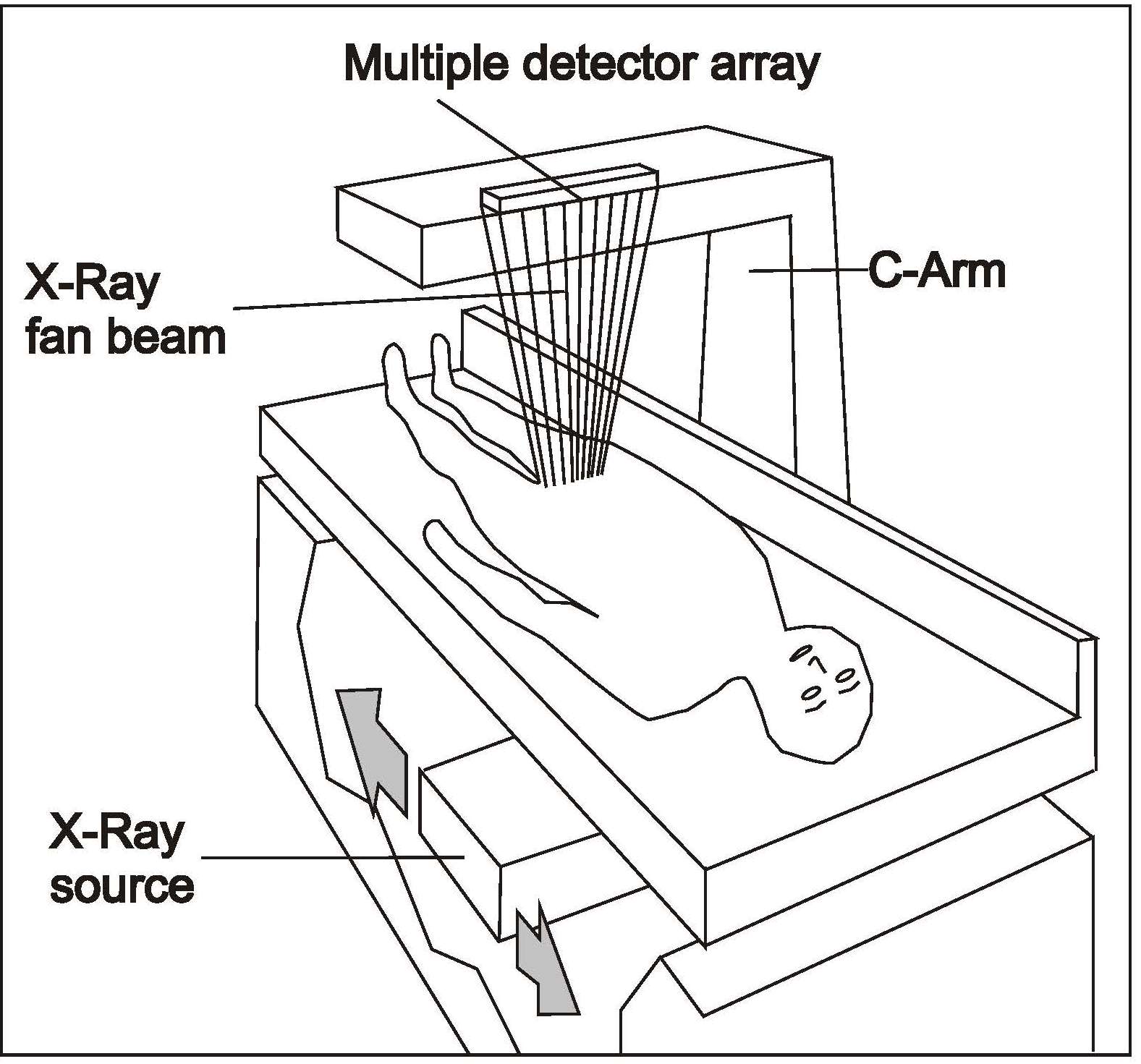
14.11.1 Applications of DXA
Dual-energy X‑ray absorptiometryis now the primary method for generating accurate data on bone mineral content and density for the diagnosis of osteoporosis (see Chapter 23 for more details). DXA is also widely used to assess body composition, with an overall precision that exceeds that of any other body composition method (Prada & Heymsfield, 2014). Both total and regional estimates of the three body compartments — fat-free mass, body fat, and bone mineral content — can be determined. However, DXA does not have the ability to discriminate between visceral, subcutaneous, and ectopic fat present in organs such as liver or muscle. Estimates of the appendicular skeletal muscle mass (ASM) can be obtained from DXA. These estimates are generated by summing the amount of lean soft tissue (LST) of the arms and legs, as they are composed mainly of muscle (except for a small amount of connective tissue and skin) (Prada & Heymsfield, 2014). From these estimates, the ASM index can be calculated by dividing the arm and leg lean soft tissue (LST) by height squared. Both the ASM and the ASM index values are considered the gold standard method for the diagnosis of sarcopenia. For published cutoff points to define sarcopenia based on DXA, see Earthman (2015). In a systematic review based on eight DXA studies by the American Society for Parenteral and Enteral Nutrition (ASPEN), DXA was recommended for the assessment of fat mass in patients with a variety of disease states, but not for fat-free mass (Sheean et al., 2020). They stressed that the validity of DXA for assessment of fat-free mass in any clinical populations remains unknown. Clearly, additional research is needed to evaluate the validity of DXA for quantifying fat-free mass in clinical populations.14.12 Ultrasound
Diagnostic ultrasound, also called sonography, is an imaging technique used in clinical settings to measure various tissue thicknesses, including muscle, bone, and subcutaneous and visceral adipose tissue. The device is portable, low cost, and capable of making fast and noninvasive regional estimates of body composition with no exposure to ionizing radiation. Consequently, this scanning technique can be used in children and pregnant women and is suitable for large epidemiological studies, although the results generated are highly dependent on the skill of the operator. The ultrasound technique involves the use of high-frequency sound waves from a transducer. These penetrate the skin surface and pass through the adipose tissue until they reach the muscle tissue. At the adipose-muscle tissue interface, a proportion of the sound waves are reflected back to the transducer as echoes. Hence, the transducer both transmits and receives the ultrasound. The degree of reflection is dependent on the changes in acoustic impedance (i.e., the product of tissue density and acoustic velocity) between two tissue interfaces. The higher the acoustic impedance, the stronger the generated reflection, and thus, the better the quality of the image. The acoustic impedance of fat and muscle are somewhat similar and much lower than bone which has a relatively high acoustic impedance; air has almost no impedance. Consequently, there is a weaker echo at the fat-muscle interface than at the muscle-bone interface. When the transducer receives the beam, it converts the echo into electric signals to form a two-dimensional image. The relative strength, or amplitude, of echoes is apparent from the brightness of the image on the computer screen, with strong reflections appearing white, weaker reflections grey, and no echoes black. As a result, a grey-scale image is produced with white borders for the skin-subcutaneous fat and muscle-bone interfaces and a visible, but less distinct border for the fat-muscle interface; see Wagner (2013) for more details. However, the interpretation of ultrasound images is difficult and depends on the technical expertise of the operator who must identify the interfaces and measure the thickness of the tissue layer of interest using electronic calipers. Care is needed to identify and place the two caliper points at the boundaries of the tissue to be measured. An automated discrimination method is available to identify the tissue boundaries for some ultrasound devices, although studies comparing the automated method with the manual discrimination are limited (Wagner, 2013). To use ultrasound, the measurement site is marked with a water-soluble transmission gel that provides acoustic contact without depression of the dermal surface. The high-resolution transducer is then placed without loss of contact with the skin so that the ultrasonic beam is perpendicular to the tissue interfaces at the marked site. A transducer receives the echoes and translates them into depth readings viewed on a computer screen. Thicknesses of 100mm or more can be measured, and density interfaces can be detected with an accuracy of 1mm. The tissue is not compressed, thereby eliminating errors associated with variations in the compressibility of skinfolds (Fanelli & Kuczmarski, 1984).14.12.1 Applications of Ultrasound
Visualization of a fetus during a prenatal examination is the most familiar application of ultrasound. However, ultrasound is also used to measure the quality and quantity of skeletal muscle mass, an index of lean soft tissue, in the elderly, patients with cystic fibrosis, and patients confined to bed rest (Prada & Heymsfield, 2014). Alternatively, measurement of the thickness of subcutaneous fat can be used to monitor changes in body composition of hospital patients receiving nutritional support. In general, the ultrasound method provides a reasonable estimate of adipose tissue thickness in humans, compared to total body electrical conductivity (TOBEC) and skinfold caliper techniques (Fanelli & Kuczmarski, 1984). For obese persons especially, ultrasound may be superior to skinfold caliper techniques for measuring subcutaneous fat (Kuczmarski et al., 1987). The method can also be used to measure thickness of other tissues such as muscle and bone, as noted earlier; for more details see Mayans et al. (2012) and Karjalainen et al. (2008). An ultrasound system has also been designed specifically for body composition which could be the user-friendly ultrasound alternative to skinfolds and other field methods for estimat;ing percentage body fat (Wagner, 2013). However, currently the diverse technology across the commercially available devices and the lack of standardized measurement protocols makes it difficult to compare results across studies (Lee et al., 2019). The American Society for Parenteral and Enteral Nutrition (ASPEN) did not recommend the use of ultrasound to assess body composition in a clinical setting, based on their systematic review of seven studies (Sheean et al., 2021). More research is needed to develop consensus on the optimal method to conduct ultrasound measurements and to generate population-specific reference data so that the method can be used to assess lean tissue and diagnose malnutrition in a clinical setting (Earthman, 2015).14.13 Summary - Method comparisons
Increasingly, clinicians are using the more robust and reproducible in vivo methods described in this chapter to measure body composition in view of the limitations of BMI as a proxy for adiposity and the recognition that differences in body composition are associated with an increased risk of diseases across the lifespan. Such diseases may include AIDS-associated wasting, diabetes, osteoporosis, sarcopenia, cardiovascular disease, and anorexia nervosa. Even during early infancy, changes in body composition (both fat and fat-free mass) can provide more understanding of the initiators and mediators of the developmental origins of adult cardiometabolic disease (McMillen et al., 2005). However, no single in vivo method has the ability to track body composition accurately from infancy to adulthood; all have strengths and technological limitations. Fields and co-workers (2015) have compared selected in vivo methods for assessing whole-body composition across the life span; details are shown in Table 14.6.Method | Infancy (< 6mo) | Childhood (2‑6y) | Adolescence (7‑17y) | Adulthood (≥ 18y) |
---|---|---|---|---|
Hydrostatic weighing | NA | I | D|I | P |
Air displacement plethysmography | P | Pa|D | P | P |
Whole-body counting for K | UE|C | UE|C | UE|C | D|C |
Dual-energy X‑ray absorptiometry | D|R | D|R | R | P|R |
Bioelectrical impedance | VA|NV | VA|NV | VA | P |
Computed tomography | UE | UE | UE | UE |
Isotope dilution | I | D|P | D|P | P |
Magnetic resonance | D|C | D|I|C | D|I|C | P|C |
Ultrasound | NV | NV | NV | NV |
- Cost of the measurement / device
- Level of patient cooperation needed for accurate results
- Feasibility in obtaining an interpretable result given the patient’s age
- Appreciation of the age of the patient relative to the assumption of the underlying principles in the chosen method.